What Is Vsc
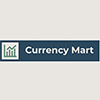
Understanding the Basics of VSC
Understanding the basics of Vehicle Stability Control (VSC) is crucial for appreciating its role in modern automotive safety. This advanced technology has become a cornerstone in vehicle design, significantly enhancing driver safety and control. To delve into the intricacies of VSC, it is essential to explore three key aspects: its definition and purpose, its historical context and development, and its key components and features. **Definition and Purpose** will provide a foundational understanding of what VSC is and why it is integrated into vehicles. This section will explain how VSC works to stabilize a vehicle during adverse driving conditions, ensuring that the vehicle remains on its intended path. **Historical Context and Development** will trace the evolution of VSC from its inception to its current state. This includes the technological advancements and regulatory changes that have driven its widespread adoption. **Key Components and Features** will dissect the technical aspects of VSC, highlighting the sensors, control units, and actuators that work in tandem to maintain vehicle stability. By examining these three facets, readers will gain a comprehensive understanding of VSC's importance and functionality. Let's begin by defining what VSC is and its purpose in modern vehicles.
Definition and Purpose
**Understanding the Basics of VSC: Definition and Purpose** Vehicle Stability Control (VSC) is a critical safety feature integrated into modern vehicles to enhance driving stability and prevent accidents. At its core, VSC is an electronic system designed to help the vehicle stay on course by automatically adjusting engine power and applying the brakes to individual wheels. This sophisticated technology works in tandem with other safety systems such as Anti-lock Braking Systems (ABS) and Traction Control Systems (TCS) to ensure optimal vehicle control. The primary purpose of VSC is to mitigate the risk of skidding or losing control, particularly during adverse driving conditions like slippery roads or sharp turns. By continuously monitoring the vehicle's speed, steering angle, and yaw rate, VSC can detect when the vehicle is deviating from its intended path. Once a discrepancy is identified, the system swiftly intervenes by reducing engine power and applying targeted braking to stabilize the vehicle. This proactive intervention helps maintain traction and prevents oversteer or understeer, thereby reducing the likelihood of a crash. In practical terms, VSC operates seamlessly in the background, often without the driver even realizing it is active. However, its presence is felt most during critical maneuvers such as emergency braking or navigating through tight corners. For instance, if a driver enters a turn too aggressively and the rear of the vehicle begins to swing out (oversteer), VSC will automatically apply gentle braking to the inside front wheel to correct the trajectory. Conversely, if the front wheels lose grip and start to slide outward (understeer), VSC will reduce engine power and apply braking to the outside rear wheel to realign the vehicle with the driver's intended path. The integration of VSC into modern vehicles has significantly improved road safety statistics. By providing an additional layer of control during unpredictable driving scenarios, VSC empowers drivers with greater confidence and peace of mind. Moreover, this technology is particularly beneficial for novice drivers who may not possess the same level of driving experience or reflexes as seasoned motorists. In essence, VSC serves as an invisible co-pilot that constantly monitors and adjusts the vehicle's dynamics to ensure safe and stable operation. In conclusion, Vehicle Stability Control is a vital component of contemporary automotive safety systems. Its definition revolves around its role in maintaining vehicle stability through automated adjustments in engine power and braking. The purpose of VSC is multifaceted: it enhances safety by preventing skids, supports drivers during challenging maneuvers, and contributes to overall road safety. As part of understanding the basics of VSC, recognizing its definition and purpose underscores its importance in modern driving environments.
Historical Context and Development
Understanding the basics of Vehicle Stability Control (VSC) requires a deep dive into its historical context and development. The concept of stability control in vehicles has its roots in the late 20th century, when automotive technology began to advance significantly. In the 1980s, the first electronic stability control systems were introduced, primarily by European manufacturers such as Bosch and Mercedes-Benz. These early systems were rudimentary but laid the groundwork for more sophisticated technologies. The real breakthrough came in the 1990s with the introduction of advanced sensors and computer algorithms that could monitor and adjust vehicle dynamics in real-time. This period saw the development of systems like Electronic Stability Program (ESP) by Bosch and Dynamic Stability Control (DSC) by BMW. These systems used a combination of sensors to detect yaw rate, lateral acceleration, and steering angle to intervene when a vehicle was about to lose stability, typically by applying the brakes to individual wheels or adjusting engine power. The widespread adoption of VSC was further accelerated by regulatory changes and consumer demand for safer vehicles. In 2007, the National Highway Traffic Safety Administration (NHTSA) in the United States mandated that all new passenger vehicles be equipped with electronic stability control by 2012. This mandate significantly pushed the industry towards universal adoption of VSC technology. Today, VSC is a standard feature in most modern vehicles, integrating seamlessly with other safety technologies such as anti-lock braking systems (ABS), traction control systems (TCS), and advanced driver assistance systems (ADAS). Modern VSC systems are highly sophisticated, leveraging advanced sensors like gyroscopes, accelerometers, and even cameras to monitor vehicle behavior. They can detect subtle changes in vehicle dynamics and make precise adjustments to maintain stability under various driving conditions. The evolution of VSC has also been influenced by advancements in software and hardware. Modern vehicles often feature advanced computer architectures that enable real-time data processing and rapid decision-making. Additionally, the integration of machine learning algorithms allows these systems to adapt to different driving styles and conditions, enhancing their effectiveness. In summary, the development of VSC is a story of continuous innovation driven by technological advancements, regulatory requirements, and consumer demand for safer vehicles. From its humble beginnings in the 1980s to its current status as a ubiquitous safety feature, VSC has played a crucial role in reducing accidents and improving road safety. Understanding this historical context provides a solid foundation for appreciating the complexities and benefits of VSC in modern vehicles.
Key Components and Features
Understanding the basics of Vehicle Stability Control (VSC) involves delving into its key components and features, which collectively ensure a vehicle's stability and safety on various road conditions. At the heart of VSC is a sophisticated network of sensors and control systems. **Sensors** play a crucial role, providing real-time data on the vehicle's speed, steering angle, yaw rate, and lateral acceleration. These sensors include wheel speed sensors, steering angle sensors, and yaw rate sensors. The **Electronic Control Unit (ECU)** processes this data to determine if the vehicle is deviating from its intended path. The ECU then communicates with the **Brake Control Module** and the **Throttle Control Module** to make necessary adjustments. When instability is detected, the VSC system can apply individual brakes to specific wheels or reduce engine power to stabilize the vehicle. This precise control is facilitated by advanced algorithms that continuously monitor and adjust the vehicle's dynamics. Another critical component is the **Actuators**, which include brake calipers and throttle valves. These actuators execute the commands from the ECU, applying gentle yet effective corrections to maintain vehicle stability. Additionally, many modern VSC systems integrate with other safety features such as **Traction Control System (TCS)** and **Anti-lock Braking System (ABS)**, enhancing overall vehicle control and safety. The **Driver Interface** is also an essential feature, often including warning lights and audible alerts that inform the driver when VSC is actively stabilizing the vehicle. This transparency helps drivers understand when their vehicle is making adjustments, fostering trust in the technology. Moreover, advanced VSC systems often include **Adaptive Features** that learn a driver's behavior over time and adjust their intervention accordingly. This adaptability ensures that the system remains unobtrusive yet effective, providing seamless support without interfering with normal driving. In summary, the key components and features of VSC—sensors, ECU, brake and throttle control modules, actuators, integration with other safety systems, driver interface, and adaptive features—work in harmony to enhance vehicle stability. By understanding these elements, drivers can appreciate the sophisticated technology that helps keep them safe on the road. This comprehensive approach to stability control underscores why VSC has become a standard feature in modern vehicles, contributing significantly to improved road safety and driving confidence.
Technical Aspects of VSC
The technical aspects of Voltage Source Converters (VSCs) are multifaceted and critical for their effective operation in various power systems. To fully understand the capabilities and applications of VSCs, it is essential to delve into three key areas: Architecture and Design, Operational Principles and Mechanisms, and Integration with Other Systems. Firstly, the **Architecture and Design** of VSCs involve the selection of appropriate topologies, such as two-level or multilevel converters, which significantly impact their performance and efficiency. Understanding these architectural choices is crucial for optimizing converter design to meet specific application requirements. Secondly, **Operational Principles and Mechanisms** explore how VSCs function at a fundamental level, including modulation techniques, control strategies, and protection mechanisms. This knowledge is vital for ensuring reliable and efficient operation under various operating conditions. Lastly, **Integration with Other Systems** highlights how VSCs interact with other components in power grids, such as renewable energy sources, energy storage systems, and transmission lines. This integration is key to leveraging the full potential of VSCs in modern power systems. By examining these aspects, we can gain a comprehensive understanding of how VSCs are designed, operate, and integrate within larger systems. Let us begin by exploring the **Architecture and Design** of VSCs, which lays the foundation for their overall functionality.
Architecture and Design
**Architecture and Design: The Technical Backbone of VSC** The architecture and design of Version Control Systems (VSC) are pivotal in ensuring the efficiency, reliability, and scalability of these critical tools. At its core, a VSC's architecture is designed to manage changes to code, documents, or other digital content over time. This involves a structured approach to organizing data, facilitating collaboration, and maintaining integrity. ### Distributed vs. Centralized Architecture One of the fundamental design choices in VSCs is between distributed and centralized architectures. **Centralized systems**, such as Subversion (SVN), rely on a single server that acts as the central repository. All users connect to this server to retrieve and commit changes. While this model is straightforward and easy to manage, it has significant drawbacks, including a single point of failure and limited scalability. On the other hand, **distributed systems**, exemplified by Git, allow each user to have a full copy of the entire history of the project. This not only enhances redundancy but also enables offline work and faster performance. ### Data Storage and Retrieval The data storage mechanism is another crucial aspect of VSC architecture. In Git, for instance, data is stored in a directed acyclic graph (DAG) structure, where each commit is a node that points to its parent commits. This structure allows for efficient branching and merging operations. Additionally, Git uses a combination of hash functions (like SHA-1) to uniquely identify commits and ensure data integrity. This ensures that any tampering with the commit history can be detected. ### Collaboration Features Effective collaboration is a key feature of modern VSCs. Design elements such as branching models (e.g., GitFlow or GitHub Flow) enable multiple developers to work on different features or bug fixes independently without interfering with each other's work. Pull requests and code reviews are integral components that facilitate peer review and ensure that changes meet certain standards before they are merged into the main branch. ### User Interface and Experience The user interface (UI) and user experience (UX) of VSC tools are also critical design considerations. A well-designed UI should be intuitive, allowing users to perform common operations like committing changes, creating branches, and resolving conflicts with ease. Tools like GitHub Desktop or GitKraken provide graphical interfaces that simplify these tasks for users who prefer not to use command-line interfaces. ### Scalability and Performance Scalability and performance are essential for large projects or enterprises. A well-architected VSC should be able to handle a high volume of commits, large file sizes, and numerous concurrent users without significant performance degradation. Techniques such as caching, load balancing, and distributed storage can help achieve this scalability. ### Security Security is a paramount concern in VSC design. Measures such as authentication (using SSH keys or tokens), authorization (via permissions and access control lists), and encryption (for data at rest and in transit) are essential to protect the integrity of the codebase. Regular updates and patches are also crucial to mitigate vulnerabilities. In summary, the architecture and design of VSCs are multifaceted and involve careful considerations of data storage, collaboration features, user interface, scalability, and security. These technical aspects collectively ensure that VSCs provide robust, reliable, and efficient tools for managing codebases across diverse projects and organizations.
Operational Principles and Mechanisms
Operational principles and mechanisms are the backbone of Voltage Source Converters (VSCs), enabling them to efficiently manage and convert electrical energy. At the core of VSCs lies the concept of pulse-width modulation (PWM), which allows for precise control over the output voltage waveform. This is achieved through the use of insulated gate bipolar transistors (IGBTs) or other power electronic devices that can be switched on and off at high frequencies. The switching pattern is determined by a control algorithm that ensures the output voltage matches the desired waveform, whether it be sinusoidal for grid applications or tailored for specific industrial processes. One of the key operational principles of VSCs is the use of a DC link capacitor, which acts as an energy buffer between the input and output stages. This capacitor helps to smooth out voltage ripples and maintain a stable DC voltage level, crucial for maintaining consistent performance. The control mechanisms involve sophisticated algorithms such as vector control, direct torque control, and model predictive control, each designed to optimize different aspects of converter operation such as efficiency, stability, and dynamic response. In grid-connected applications, VSCs must adhere to stringent standards regarding power quality and grid stability. Here, operational mechanisms include synchronization techniques like phase-locked loops (PLLs) to ensure that the converter's output is synchronized with the grid frequency and phase. Additionally, advanced control strategies such as droop control and virtual inertia emulation are employed to enhance grid stability and support during disturbances. The modular multilevel converter (MMC) is another significant operational mechanism within VSCs, particularly in high-voltage direct current (HVDC) systems. The MMC architecture allows for scalability and redundancy by using multiple sub-modules connected in series and parallel configurations. Each sub-module contains a half-bridge or full-bridge converter that can be controlled independently, enabling fine-grained control over the output voltage waveform and reducing harmonic distortion. In terms of technical aspects, the selection of semiconductor devices is critical for VSC performance. Modern VSCs often utilize wide bandgap semiconductors like silicon carbide (SiC) or gallium nitride (GaN), which offer higher switching frequencies, lower losses, and improved thermal management compared to traditional silicon-based devices. Furthermore, advancements in digital signal processing and real-time computing have enabled more sophisticated control algorithms that can adapt to changing operational conditions in real-time. Overall, the operational principles and mechanisms of VSCs are designed to provide high efficiency, reliability, and flexibility across a wide range of applications from renewable energy integration to industrial drives and power transmission systems. By leveraging advanced control strategies, modular architectures, and cutting-edge semiconductor technologies, VSCs continue to play a pivotal role in modern power systems.
Integration with Other Systems
### Integration with Other Systems The integration of Vehicle Stability Control (VSC) with other vehicle systems is a critical aspect of its functionality and effectiveness. VSC does not operate in isolation but rather as part of a comprehensive suite of safety and control technologies. One key integration is with the Anti-lock Braking System (ABS), where VSC leverages ABS's ability to control individual wheel braking to stabilize the vehicle during cornering or sudden maneuvers. This synergy allows VSC to apply precise braking to specific wheels, helping to correct the vehicle's trajectory and maintain stability. Another crucial integration is with the Electronic Throttle Control System (ETC), which enables VSC to adjust engine power output in real-time. By reducing or increasing engine torque, VSC can help stabilize the vehicle by managing the amount of power delivered to the wheels. This dynamic adjustment ensures that the vehicle remains stable and responsive, even under adverse driving conditions. Additionally, VSC often integrates with Traction Control Systems (TCS), which monitor and control wheel spin. When TCS detects excessive wheel spin, it can work in tandem with VSC to reduce engine power and apply selective braking, thereby enhancing traction and stability. This combined effort prevents wheels from losing grip, especially on slippery or uneven surfaces. The integration with Electronic Stability Control (ESC) systems is also noteworthy. While ESC and VSC share similar goals, they operate under different conditions; ESC focuses on stabilizing the vehicle during loss of traction, whereas VSC is more focused on maintaining stability during cornering or sudden maneuvers. However, their combined operation ensures a holistic approach to vehicle stability, covering a broader range of driving scenarios. Furthermore, modern vehicles often feature advanced driver assistance systems (ADAS) such as Lane Departure Warning (LDW) and Adaptive Cruise Control (ACC). These systems can share data with VSC to enhance its predictive capabilities. For instance, if LDW detects that the vehicle is drifting out of its lane, it can alert VSC to prepare for potential corrective actions, thereby improving response times and overall vehicle stability. In terms of data exchange, VSC relies on a network of sensors and actuators that communicate through high-speed data buses like CAN (Controller Area Network) or FlexRay. These networks enable real-time data sharing between various control units, allowing VSC to receive inputs from sensors such as gyroscopes, accelerometers, and wheel speed sensors. This seamless communication ensures that VSC can react swiftly and accurately to changes in the vehicle's dynamics. In conclusion, the integration of VSC with other vehicle systems is essential for its optimal performance. By leveraging the capabilities of ABS, ETC, TCS, ESC, and ADAS, VSC can provide comprehensive stability control that enhances safety and driving confidence. This multifaceted approach underscores the sophisticated engineering behind modern vehicle safety technologies and highlights the importance of system integration in achieving superior vehicle performance and safety.
Applications and Benefits of VSC
The applications and benefits of Variable Speed Controllers (VSCs) are multifaceted and far-reaching, impacting various sectors in significant ways. At the heart of modern industrial and commercial operations, VSCs offer unparalleled control over machinery, enhancing efficiency and reducing energy consumption. Beyond their immediate practical uses, VSCs also contribute to environmental sustainability by minimizing waste and optimizing resource allocation. Economically, they provide substantial savings through reduced energy costs and extended equipment lifespan. Looking ahead, future trends and innovations in VSC technology promise even greater advancements, such as integration with AI and IoT systems to further optimize performance. This article delves into the industrial and commercial uses of VSCs, exploring how these devices are revolutionizing manufacturing processes and operational efficiency. By examining the current applications and future potential of VSCs, we can better understand their transformative impact on industries worldwide. Let us begin by exploring the industrial and commercial uses of VSCs, where their benefits are most pronounced.
Industrial and Commercial Uses
Industrial and commercial applications of Voltage Source Converters (VSCs) are diverse and pivotal, driving efficiency, reliability, and innovation across various sectors. In the realm of power transmission and distribution, VSCs are integral to High Voltage Direct Current (HVDC) systems, enabling the efficient transfer of power over long distances with minimal energy loss. This is particularly beneficial for connecting remote renewable energy sources to the main grid, thereby enhancing the integration of wind and solar power into the energy mix. For instance, offshore wind farms often rely on VSC-based HVDC systems to transmit electricity back to the mainland, ensuring stable and efficient power delivery. In industrial settings, VSCs are crucial for motor drives and adjustable speed drives, allowing for precise control over motor speed and torque. This capability is essential in applications such as steel mills, paper mills, and textile manufacturing, where variable speed control can significantly improve process efficiency and reduce energy consumption. Additionally, VSCs are used in active front-end (AFE) drives for industrial power supplies, providing high power factor correction and reducing harmonic distortion, which helps in maintaining grid stability and compliance with regulatory standards. Commercial buildings also leverage VSC technology for advanced power quality solutions. Uninterruptible Power Supplies (UPS) systems, which are vital for data centers and other mission-critical facilities, often employ VSCs to ensure continuous power supply during grid outages. These systems provide clean, stable power to sensitive electronic equipment, safeguarding against data loss and downtime. Furthermore, VSCs are used in building automation systems to manage energy distribution efficiently, optimizing energy usage through smart grid technologies. The benefits of VSCs in industrial and commercial contexts extend beyond operational efficiency. They offer enhanced reliability by providing fault tolerance and redundancy, which are critical in high-availability environments. The modular design of many VSC systems allows for easy maintenance and scalability, reducing downtime and enabling seamless upgrades as operational needs evolve. Moreover, the advanced control algorithms embedded in modern VSCs enable real-time monitoring and predictive maintenance, helping to prevent potential issues before they occur. In summary, the industrial and commercial uses of VSCs underscore their versatility and importance in modern power systems. From enabling efficient long-distance power transmission to providing precise control over industrial processes and ensuring high-quality power supply in commercial settings, VSCs play a vital role in enhancing operational efficiency, reliability, and sustainability. As technology continues to evolve, the applications and benefits of VSCs are likely to expand further, driving innovation and excellence across various industries.
Environmental and Economic Advantages
The integration of Voltage Source Converters (VSCs) into modern power systems offers a myriad of environmental and economic advantages, significantly enhancing the efficiency and sustainability of energy transmission and distribution. From an environmental perspective, VSCs play a crucial role in facilitating the integration of renewable energy sources such as wind and solar power into the grid. By enabling the efficient conversion of DC power from these sources to AC power, VSCs help reduce reliance on fossil fuels, thereby decreasing greenhouse gas emissions and contributing to a cleaner environment. Additionally, VSCs can improve grid stability and resilience, which is essential for maintaining a reliable supply of electricity as the share of intermittent renewable sources increases. Economically, the deployment of VSCs can lead to substantial cost savings. For instance, in high-voltage direct current (HVDC) transmission systems, VSCs allow for more efficient transmission over long distances with lower energy losses compared to traditional AC systems. This not only reduces operational costs but also minimizes the need for additional infrastructure, such as substations and transmission lines. Furthermore, VSCs enable the implementation of advanced grid management strategies, including real-time power flow control and reactive power compensation, which can optimize energy usage and reduce peak demand charges. This capability is particularly beneficial in smart grid applications where dynamic pricing and demand response mechanisms are employed to manage energy consumption more effectively. Moreover, the flexibility of VSCs in handling power flow control allows for better utilization of existing grid assets, delaying the need for costly upgrades or new infrastructure investments. This flexibility also extends to the ability to provide ancillary services like voltage support and frequency regulation, which are critical for maintaining grid stability and can be monetized through various market mechanisms. In summary, the environmental and economic advantages of VSCs make them a vital component in the transition towards a more sustainable and efficient energy system, offering significant benefits in terms of reduced carbon footprint, improved grid reliability, and cost savings. As the world continues to move towards a cleaner and more interconnected energy landscape, the role of VSCs will only become more pivotal in ensuring a resilient and economically viable power grid.
Future Trends and Innovations
As we delve into the applications and benefits of Voltage Source Converters (VSCs), it is imperative to explore the future trends and innovations that are set to revolutionize their use. The landscape of power electronics is evolving rapidly, driven by advancements in semiconductor technology, artificial intelligence, and the increasing demand for sustainable energy solutions. One of the most significant trends is the integration of VSCs with renewable energy sources such as solar and wind power. Next-generation VSCs are being designed with enhanced capabilities to handle the variable output of these sources, ensuring grid stability and efficiency. For instance, the development of modular multilevel converters (MMC) has significantly improved the scalability and reliability of VSCs, making them ideal for high-voltage direct current (HVDC) transmission systems. Another key innovation is the adoption of wide bandgap (WBG) semiconductors like silicon carbide (SiC) and gallium nitride (GaN). These materials offer superior performance characteristics compared to traditional silicon-based devices, including higher switching frequencies, lower losses, and increased thermal tolerance. This shift is expected to enhance the efficiency and compactness of VSCs, enabling their deployment in a broader range of applications from electric vehicles to industrial power supplies. The integration of advanced control algorithms and machine learning techniques is also transforming the field. Real-time monitoring and predictive maintenance enabled by IoT technologies will allow for more efficient operation and reduced downtime of VSC systems. Additionally, the incorporation of blockchain technology could facilitate secure peer-to-peer energy trading in decentralized grids, further democratizing access to renewable energy. Furthermore, there is a growing focus on grid resilience and flexibility. Future VSCs will be designed to support microgrids and smart grids, enabling seamless transition between different power sources and ensuring continuous supply even during outages. This adaptability will be crucial as grids become more complex and dynamic. In terms of sustainability, innovations in VSC design are aimed at reducing environmental impact. For example, the use of recyclable materials and the development of more energy-efficient cooling systems are becoming priorities. Moreover, advancements in power-to-X (P2X) technologies—where electricity is converted into other forms of energy such as hydrogen or synthetic fuels—will leverage VSCs to create a more circular economy. Lastly, the convergence of VSC technology with other emerging fields like electric vehicles and energy storage systems is expected to drive significant growth. As electric vehicles become more prevalent, VSCs will play a critical role in fast-charging infrastructure and vehicle-to-grid (V2G) applications. Similarly, advancements in battery storage will be complemented by sophisticated VSCs that optimize charging/discharging cycles and ensure grid stability. In conclusion, the future trends and innovations surrounding VSCs are poised to revolutionize various sectors by enhancing efficiency, sustainability, and resilience. As these technologies continue to evolve, they will not only support the widespread adoption of renewable energy but also pave the way for a smarter, more interconnected world.