What Does The Universe Look Like
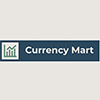
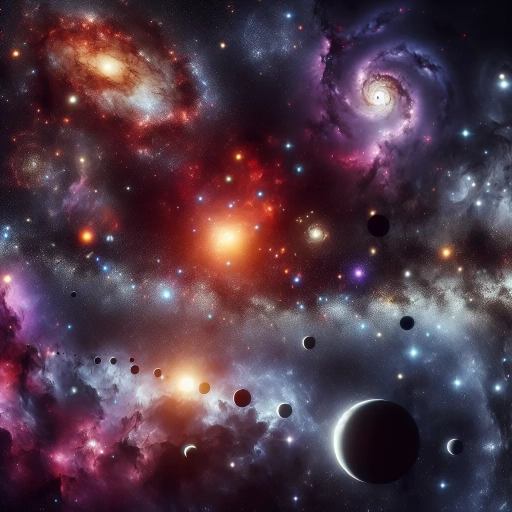
The universe, a vast and intricate expanse, has long fascinated humanity with its mysteries and wonders. Understanding its structure and evolution is a complex yet captivating endeavor. This article delves into the multifaceted nature of the universe, exploring three key aspects: the observable universe, the large-scale features that define its architecture, and the early formation and evolution that shaped it into what we see today. We begin by examining **The Observable Universe**, which encompasses all that we can see and study, setting the stage for a deeper exploration of its grand design and historical development. This foundational understanding will then lead us to discuss **The Universe's Large-Scale Features**, including galaxies, galaxy clusters, and superclusters, which reveal the universe's hierarchical structure. Finally, we will delve into **The Universe's Early Formation and Evolution**, tracing back to the Big Bang and the subsequent processes that have sculpted the cosmos over billions of years. By starting with **The Observable Universe**, we establish a clear framework for appreciating the broader tapestry of the cosmos.
The Observable Universe
The observable universe, the vast expanse of space that we can see, is a complex and fascinating realm that has captivated human curiosity for centuries. This article delves into three key aspects that illuminate our understanding of this cosmic landscape: Galactic Structures and Clusters, Cosmic Microwave Background Radiation, and Distant Quasars and Galaxies. By examining the intricate networks of galaxies and galaxy clusters, we gain insights into the large-scale structure of the universe. The Cosmic Microwave Background Radiation provides a snapshot of the universe's earliest moments, offering clues about its origins and evolution. Meanwhile, observations of distant quasars and galaxies allow us to peer into the universe's distant past, revealing how galaxies formed and evolved over billions of years. Each of these areas of study contributes to a richer understanding of the observable universe, starting with the foundational knowledge of Galactic Structures and Clusters, which form the backbone of cosmic organization and set the stage for further exploration.
Galactic Structures and Clusters
Galactic structures and clusters are fundamental components of the observable universe, offering insights into its vast and intricate architecture. Galaxies, the building blocks of these structures, come in various shapes and sizes, including spiral, elliptical, and irregular types. Spiral galaxies like the Milky Way feature a central bulge surrounded by a disk of stars, gas, and dust, while elliptical galaxies are more spherical and contain older stars. Irregular galaxies lack a distinct shape due to interactions with other galaxies. Galactic clusters are groups of galaxies bound together by gravity. These clusters can range from small groups of a few galaxies to massive galaxy clusters containing thousands of galaxies. The Coma Cluster, for instance, is one of the largest known galaxy clusters in the observable universe, spanning over 20 million light-years across and containing more than 1,000 galaxies. These clusters are not isolated entities but are part of larger superclusters that form vast networks across the cosmos. Superclusters are the largest known structures in the universe, comprising numerous galaxy clusters and superclusters themselves. The Sloan Great Wall, discovered in 2003, is one such supercluster that stretches over 1.37 billion light-years across, making it one of the largest known structures in the observable universe. These superclusters are separated by vast voids where few galaxies exist, creating a web-like pattern known as the cosmic web. The formation and evolution of these galactic structures are influenced by dark matter and dark energy, which play crucial roles in shaping the universe's large-scale structure. Dark matter provides the gravitational scaffolding for normal matter to clump together into galaxies and galaxy clusters, while dark energy drives the accelerating expansion of the universe. Observational evidence from telescopes and space missions like the Hubble Space Telescope and the Sloan Digital Sky Survey has significantly advanced our understanding of galactic structures and clusters. These observations reveal that galaxies within clusters are often moving at high speeds relative to each other and that some clusters are still in the process of forming through mergers with smaller groups. Understanding galactic structures and clusters not only provides a glimpse into the universe's current state but also offers clues about its past and future evolution. The study of these structures helps cosmologists refine models of the universe's origins and expansion, ultimately contributing to a more comprehensive picture of what the universe looks like on its grandest scales.
Cosmic Microwave Background Radiation
The Cosmic Microwave Background Radiation (CMB) is a pivotal component in understanding the observable universe, offering a snapshot of the universe's earliest moments. Discovered in 1964 by Arno Penzias and Robert Wilson, the CMB is the residual heat from the Big Bang, detectable in the form of microwave radiation that permeates the cosmos. This radiation is a remnant of the epoch when the universe had cooled enough for electrons and protons to combine into neutral atoms, a period known as recombination, occurring approximately 380,000 years after the Big Bang. The CMB's uniformity, with tiny fluctuations of about one part in 100,000, provides crucial evidence for the Big Bang theory and serves as a fundamental tool for cosmologists to study the universe's structure and evolution. These fluctuations are thought to be the seeds of galaxy formation, as they represent variations in density that eventually led to the gravitational collapse of matter into galaxies and galaxy clusters. The CMB's blackbody spectrum, consistent with a temperature of about 2.725 degrees Kelvin, further supports its origin from the early universe. Observations by satellites such as COBE, WMAP, and Planck have mapped the CMB with increasing precision, revealing details about the universe's composition, geometry, and the properties of dark matter and dark energy. The study of the CMB continues to be a cornerstone of modern cosmology, providing insights into the universe's origins and its current state, thereby enhancing our understanding of the observable universe.
Distant Quasars and Galaxies
Distant quasars and galaxies offer a glimpse into the early universe, providing valuable insights into its evolution and structure. Quasars, incredibly luminous objects believed to be powered by supermassive black holes at the centers of galaxies, are among the most distant observable objects. These ancient beacons emit massive amounts of energy across the electromagnetic spectrum, making them visible from billions of light-years away. By studying quasars, scientists can infer the presence of massive galaxies in the distant past, often during the epoch of reionization when the first stars and galaxies began to form. Galaxies, too, are crucial for understanding cosmic history. Observations of distant galaxies reveal how they have changed over billions of years. The Hubble Space Telescope and other advanced telescopes have captured images of galaxies in various stages of evolution, from compact, star-forming galaxies in the early universe to the more structured spiral and elliptical galaxies seen today. These observations suggest that many modern galaxies have undergone significant mergers and interactions, leading to their current forms. The light from these distant objects is often shifted towards the red end of the spectrum due to cosmic expansion—a phenomenon known as redshift. By measuring this redshift, astronomers can determine how far away these objects are and how long ago their light was emitted. This method allows scientists to construct a timeline of the universe's history, tracing back to the Big Bang. Furthermore, the study of distant quasars and galaxies helps in understanding the intergalactic medium (IGM), the diffuse gas that fills the vast spaces between galaxies. The absorption lines seen in quasar spectra provide clues about the composition and temperature of this gas, which played a critical role in the formation of stars and galaxies. In addition, observations of distant quasars and galaxies have implications for cosmology. They help constrain models of dark matter and dark energy, which are thought to dominate the universe's mass-energy budget but remain invisible. The distribution and properties of these distant objects can also be used to test theories of gravity on large scales. Overall, distant quasars and galaxies serve as cosmic time capsules, offering a window into the universe's past. By studying these objects, scientists gain a deeper understanding of how the universe evolved from its earliest moments to its current state, enriching our knowledge of the observable universe.
The Universe's Large-Scale Features
The universe, with its vast expanse and intricate structures, is a subject of profound fascination and ongoing scientific inquiry. Understanding its large-scale features requires delving into several key aspects. The early formation and evolution of the universe, as described by the Big Bang Theory, set the stage for the cosmos as we know it today. This period includes the initial conditions that governed the universe's expansion, primordial nucleosynthesis which formed the first elements, and the era of reionization that saw the emergence of galaxies. Additionally, the role of dark matter and dark energy is crucial in shaping the universe's structure and driving its accelerating expansion. These elements collectively contribute to the formation of superclusters and filaments, which are the largest known structures in the universe, weaving together galaxies and galaxy clusters into a cosmic web. By examining these interconnected themes, we gain a deeper insight into the universe's grand architecture and its dynamic evolution over billions of years. Transitioning from these foundational concepts, we can explore how superclusters and filaments are the ultimate manifestation of these processes, forming the backbone of the universe's large-scale structure.
Superclusters and Filaments
Superclusters and filaments are among the most striking large-scale features of the universe, revealing its intricate and interconnected structure. **Superclusters** are vast networks of galaxy clusters and superclusters that stretch across millions of light-years. These massive agglomerations are not isolated entities but are part of an even larger web-like structure known as the cosmic web. Within superclusters, galaxies are grouped into clusters, which in turn are linked by filaments—long, thread-like structures composed of dark matter and gas. These filaments act as cosmic highways, guiding the flow of matter and energy across the universe. **Filaments** are the backbone of the cosmic web, forming a complex network that crisscrosses the universe. They are regions of higher density compared to the surrounding voids, where galaxies and galaxy clusters tend to congregate. These filaments can be hundreds of millions of light-years long and are thought to be held together by dark matter, which provides the gravitational scaffolding necessary for their formation. The distribution of galaxies within these filaments is not random; instead, they often align along these structures, creating a pattern that reflects the underlying distribution of dark matter. The study of superclusters and filaments has provided significant insights into the evolution and structure of the universe. For instance, observations from surveys like the Sloan Digital Sky Survey (SDSS) have mapped out extensive networks of filaments and superclusters, highlighting their role in shaping galaxy distributions on a cosmic scale. These structures are also crucial for understanding how galaxies form and evolve over billions of years, as they influence the flow of gas and matter that fuels star formation. Moreover, superclusters and filaments offer a window into the early universe. Simulations based on the Lambda-CDM model of cosmology predict that these large-scale structures formed through gravitational collapse from tiny fluctuations in the density of the universe shortly after the Big Bang. The observation of these structures today confirms many aspects of this model, providing strong evidence for our current understanding of cosmic evolution. In summary, superclusters and filaments represent some of the most fascinating aspects of the universe's large-scale features. They underscore the interconnected nature of cosmic structures and highlight the role of dark matter in shaping these vast networks. By studying these phenomena, scientists gain deeper insights into the universe's history, evolution, and underlying physical laws, ultimately enriching our understanding of what the universe looks like on its grandest scales.
The Role of Dark Matter and Dark Energy
The Universe's Early Formation and Evolution
The Big Bang Theory and Initial Conditions
Primordial Nucleosynthesis and Element Formation
The Era of Reionization and Galaxy Formati
The universe's large-scale features are intricately linked to the roles of dark matter and dark energy, which have been pivotal in shaping its evolution since the Big Bang. Dark matter, an invisible form of matter that does not emit, absorb, or reflect light, played a crucial role in the early formation of galaxies. During the era of reionization, dark matter provided the gravitational scaffolding necessary for normal matter to clump together and form the first stars and galaxies. This process was essential for the subsequent formation of larger galaxy clusters and superclusters, which are the building blocks of the universe's large-scale structure. Dark energy, on the other hand, has dominated the universe's expansion in recent epochs. Discovered through observations of distant supernovae and confirmed by other lines of evidence such as cosmic microwave background radiation and large-scale structure surveys, dark energy is thought to be responsible for the accelerating expansion of the universe. This acceleration has significant implications for the future of cosmic evolution, as it suggests that galaxies will continue to move away from each other at an ever-increasing rate. The interplay between dark matter and dark energy is critical for understanding how the universe transitioned from a homogeneous, dense state immediately after the Big Bang to its current diverse and expansive form. Primordial nucleosynthesis, which occurred within the first few minutes after the Big Bang, set the stage for element formation by creating light elements such as hydrogen, helium, and lithium. However, it was dark matter that facilitated the gravitational collapse of these elements into denser regions, eventually leading to the formation of stars and galaxies. The era of reionization marked a significant phase where these early galaxies began to ionize the surrounding intergalactic medium, paving the way for further galaxy formation and evolution. Today, we observe a universe with vast galaxy clusters and superclusters separated by enormous voids—a structure that could not have emerged without the influence of dark matter and dark energy. Understanding these components is essential for a comprehensive view of what the universe looks like on its largest scales, highlighting how these mysterious entities have sculpted the cosmos into its current grand tapestry.
The Role of Dark Matter and Dark Energy
The universe's large-scale features are significantly influenced by two mysterious components: dark matter and dark energy. Dark matter, which constitutes approximately 27% of the universe's total mass-energy density, plays a crucial role in the formation and structure of galaxies and galaxy clusters. It does not emit, absorb, or reflect any electromagnetic radiation, making it invisible to our telescopes, yet its presence is evident through its gravitational effects. For instance, the rotation curves of galaxies indicate that stars and gas are moving at a constant velocity, suggesting that there is a large amount of unseen mass holding these systems together. Additionally, the distribution of galaxy clusters and the large-scale structure of the universe can be explained by the presence of dark matter, which provides the necessary gravitational scaffolding for normal matter to clump together. Dark energy, on the other hand, accounts for about 68% of the universe's mass-energy density and is responsible for the accelerating expansion of the universe. Discovered in the late 1990s through observations of distant supernovae, dark energy acts as a negative pressure that pushes matter apart, causing galaxies to move away from each other at an ever-increasing rate. This phenomenon is particularly evident in the redshift of light from distant galaxies and the cosmic microwave background radiation. The interplay between dark matter and dark energy shapes the universe's large-scale features; while dark matter helps in forming and stabilizing structures like galaxies and galaxy clusters, dark energy drives their separation over vast distances. Understanding these components is essential for a comprehensive view of the universe's evolution and current state. The balance between dark matter's gravitational pull and dark energy's expansive force determines how galaxies and galaxy clusters form and evolve over billions of years. Furthermore, studying these components can provide insights into fundamental questions about the nature of gravity, the properties of matter and energy, and ultimately, the fate of the universe itself. Despite their elusive nature, dark matter and dark energy are indispensable elements in our understanding of cosmic structure and evolution, highlighting the intricate and complex dynamics that govern the universe's large-scale features.
The Universe's Early Formation and Evolution
The formation and evolution of the universe is a complex and fascinating topic, deeply rooted in several key scientific theories and processes. At its core, our understanding begins with **The Big Bang Theory and Initial Conditions**, which describe the universe's origin and the initial conditions that set the stage for its development. This foundational concept is complemented by **Primordial Nucleosynthesis and Element Formation**, which explains how the first elements were created in the universe's early moments. Additionally, **The Era of Reionization and Galaxy Formation** sheds light on how the universe transitioned from a dark, neutral state to one filled with luminous galaxies. These three pillars of knowledge collectively provide a comprehensive framework for understanding the universe's early formation and evolution. By delving into these areas, we can gain a deeper insight into the cosmos's history and its current state. Let us start by exploring **The Big Bang Theory and Initial Conditions**, which serve as the fundamental basis for all subsequent developments in the universe's history.
The Big Bang Theory and Initial Conditions
The Big Bang Theory, the cornerstone of modern cosmology, posits that the universe began as an infinitely hot and dense point approximately 13.8 billion years ago. This singular event marked the inception of space, time, and matter as we know it. The initial conditions of this primordial state are crucial for understanding the universe's early formation and evolution. At the Big Bang, all matter was condensed into a singularity, with temperatures and densities so extreme that the laws of physics as we understand them today did not apply. As this singularity expanded, it cooled, allowing for the formation of subatomic particles such as protons, neutrons, and electrons. This period, known as the Quark Epoch, transitioned into the Hadron Epoch, where protons and neutrons coalesced into atomic nuclei. The universe continued to expand and cool, eventually reaching a point where electrons and nuclei combined to form neutral atoms, primarily hydrogen and helium, in an era known as the Recombination Epoch. These initial conditions set the stage for the subsequent formation of galaxies, stars, and eventually, the complex structures we observe in the universe today. The precise balance of these early conditions—such as the ratio of matter to radiation and the presence of tiny fluctuations in density—was critical for the universe's evolution into its current state, highlighting the intricate interplay between fundamental physics and cosmic history. Understanding these initial conditions through observations of cosmic microwave background radiation and large-scale structure provides valuable insights into the universe's origins and its ongoing evolution.
Primordial Nucleosynthesis and Element Formation
Primordial nucleosynthesis, occurring in the first 20 minutes after the Big Bang, is a critical phase in the universe's early formation and evolution. During this period, the universe was still extremely hot and dense, with temperatures exceeding billions of degrees. Under these conditions, protons, neutrons, and electrons began to combine into the lightest elements. The process started when the universe cooled enough for protons and neutrons to form deuterons (a proton-neutron pair), marking the beginning of nucleosynthesis. As the universe continued to expand and cool, deuterons combined to form helium-3 and helium-4, along with trace amounts of lithium-7 and beryllium-7. This era ended when the universe had cooled sufficiently for nuclear reactions to cease, leaving behind a primordial abundance of hydrogen (~75% by mass), helium (~25% by mass), and trace amounts of other light elements. This period is pivotal because it sets the stage for subsequent element formation processes. The light elements produced during primordial nucleosynthesis served as the building blocks for heavier elements formed later through stellar nucleosynthesis. Stars, which began forming around 100 million years after the Big Bang, fused these light elements into heavier ones like carbon, nitrogen, oxygen, and iron through nuclear reactions in their cores. When these stars eventually exploded as supernovae, they dispersed these heavier elements throughout the universe, enriching interstellar gas and enabling the formation of planets and life. Understanding primordial nucleosynthesis also provides insights into the universe's fundamental properties. The abundance of light elements produced during this era is highly sensitive to the universe's baryon-to-photon ratio and the number of neutrino species present. Observations of these elemental abundances, particularly deuterium and helium-4, have been used to constrain models of the universe's early conditions and validate the Big Bang theory. Furthermore, discrepancies between predicted and observed abundances have led to refinements in our understanding of cosmological parameters and the role of dark matter and dark energy in the universe's evolution. In summary, primordial nucleosynthesis is a cornerstone of cosmology, bridging the gap between the universe's inception and its current state. It not only explains the origin of light elements but also underpins our understanding of subsequent stellar and planetary formation processes. The study of this era continues to be a vibrant area of research, offering a window into the universe's earliest moments and informing our broader comprehension of cosmic evolution.
The Era of Reionization and Galaxy Formation
The Era of Reionization and Galaxy Formation marks a pivotal phase in the early universe, occurring approximately 13 billion years ago. During this period, the universe transitioned from a dark, neutral state to one where light could travel freely, and galaxies began to take shape. Initially, after the Big Bang, the universe was filled with neutral hydrogen gas that absorbed and scattered light, making it opaque. However, as the first stars and galaxies formed, they emitted intense ultraviolet radiation that ionized the surrounding hydrogen, creating bubbles of ionized gas. Over time, these bubbles expanded and merged, eventually reionizing the entire universe. This era was crucial for galaxy formation because it allowed for the collapse of gas clouds under gravity, leading to the birth of new stars and the growth of galaxies. The process was complex, with feedback mechanisms from star formation and supernovae explosions regulating the rate at which galaxies grew. Early galaxies were likely small and irregular, but through mergers and accretion of gas, they evolved into the diverse range of galaxy types we see today. Observational evidence from telescopes like the Hubble Space Telescope and the Atacama Large Millimeter/submillimeter Array (ALMA) has provided insights into this era. The detection of distant quasars and galaxies in the early universe suggests that reionization was likely a patchy process, with some regions becoming ionized before others. Simulations also support this view, indicating that reionization was driven by a combination of stellar and black hole activity. Understanding the Era of Reionization and Galaxy Formation is essential for piecing together the history of the universe. It highlights how the interplay between matter and radiation shaped the cosmos into its current form. This period laid the groundwork for the subsequent evolution of galaxies, including our own Milky Way, and continues to influence our understanding of cosmic structure and the distribution of matter on large scales. As new observational and computational tools become available, scientists are refining their models of this critical epoch, offering a clearer picture of how our universe came to be as we see it today.