What Is Deposition Science Definition
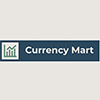
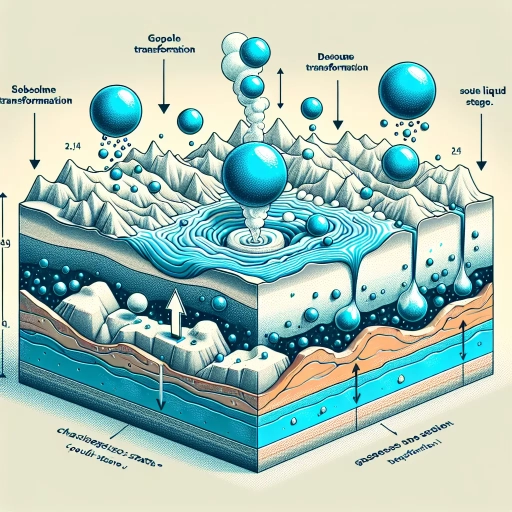
Deposition science is a multifaceted field that encompasses the study of how materials are deposited onto surfaces, forming layers or films. This discipline is crucial in various technological and industrial applications, from semiconductor manufacturing to biomedical engineering. At its core, deposition science involves understanding the basic concepts that govern the deposition process, including the physical and chemical principles that dictate how materials adhere to surfaces. Delving deeper, it is essential to explore the processes and mechanisms involved in deposition, such as chemical vapor deposition (CVD) and physical vapor deposition (PVD), which are pivotal in controlling the properties of the deposited materials. Furthermore, the applications and implications of deposition science are vast, influencing fields like electronics, energy storage, and medical devices. This article will provide a comprehensive overview of deposition science, starting with an exploration of its basic concepts to lay the foundation for a deeper understanding of its processes and applications. To begin, let's delve into **Understanding Deposition Science: Basic Concepts**.
Understanding Deposition Science: Basic Concepts
Understanding Deposition Science is a multifaceted field that encompasses a broad range of concepts and historical developments. At its core, Deposition Science involves the study of how materials are deposited onto surfaces, a process crucial in various industrial and natural environments. To grasp this complex subject, it is essential to delve into its **Definition and Scope**, which outlines the fundamental principles and applications of deposition science. This foundation is built upon a rich **Historical Development and Key Milestones**, highlighting significant discoveries and advancements that have shaped the field over time. Additionally, the **Interdisciplinary Nature of Deposition Science** underscores how it intersects with physics, chemistry, biology, and engineering, making it a dynamic and versatile area of study. By exploring these aspects, one can gain a comprehensive understanding of deposition science. Let us begin by examining the **Definition and Scope of Deposition Science**, which provides the groundwork for understanding this intricate field.
Definition and Scope of Deposition Science
Deposition science, a multidisciplinary field, encompasses the study of processes and mechanisms by which materials are deposited onto surfaces. This scientific discipline is crucial in various industrial, technological, and environmental contexts. At its core, deposition science involves understanding the chemical, physical, and biological interactions that lead to the formation of layers or films on substrates. The scope of deposition science is broad, spanning across different methods such as chemical vapor deposition (CVD), physical vapor deposition (PVD), electrochemical deposition, and biological deposition. Chemical vapor deposition (CVD) involves the reaction of gaseous precursors to form a solid material on a substrate, commonly used in semiconductor manufacturing and nanotechnology. Physical vapor deposition (PVD) includes techniques like sputtering and evaporation, where solid materials are vaporized and then condensed onto a surface, often applied in thin film coatings for electronics and optics. Electrochemical deposition, or electroplating, utilizes an electric current to deposit ions from a solution onto an electrode, widely employed in metal finishing and battery production. Biological deposition, on the other hand, involves natural processes such as the formation of biofilms by microorganisms or the accumulation of organic matter in sediments. This aspect is vital in fields like microbiology, ecology, and environmental science. The principles of deposition science are also integral to understanding geological phenomena such as sedimentation and mineral formation. In addition to these methods, deposition science delves into the properties and applications of deposited materials. For instance, it explores how deposited layers can enhance surface properties like conductivity, hardness, or biocompatibility. The field also addresses issues related to scalability, uniformity, and the environmental impact of deposition processes. Understanding deposition science is essential for advancing technologies in fields such as energy storage (e.g., batteries), electronics (e.g., semiconductor devices), biomedical engineering (e.g., implants), and environmental remediation (e.g., water treatment). By elucidating the fundamental mechanisms and optimizing deposition techniques, researchers and engineers can develop innovative materials and applications that drive technological progress and solve real-world problems. Thus, the definition and scope of deposition science highlight its critical role in bridging basic scientific principles with practical technological innovations.
Historical Development and Key Milestones
The historical development of deposition science is a rich and multifaceted narrative that spans centuries, marked by significant milestones that have shaped our understanding of this fundamental process. The earliest recorded observations of deposition date back to ancient civilizations, where natural phenomena such as sedimentation in rivers and the formation of stalactites and stalagmites in caves were noted. However, it wasn't until the 17th and 18th centuries that scientific inquiry into these processes began to take shape. One key milestone was the work of James Hutton in the late 18th century, who is often credited with laying the foundations of modern geology. Hutton's theory of uniformitarianism posited that the Earth's features were shaped by slow, continuous processes over long periods, which included deposition. This concept revolutionized the field by providing a framework for understanding how sediments accumulate and form new rock layers. The 19th century saw further advancements with the development of plate tectonics, which explained how continents move and interact, influencing deposition patterns. Charles Lyell's work on stratigraphy and the principle of superposition—where older layers are buried beneath younger ones—provided crucial tools for interpreting geological history through deposited sediments. In the 20th century, technological advancements allowed for more precise studies. The invention of radiometric dating techniques enabled scientists to accurately determine the age of deposited materials, while seismic surveys and deep-sea drilling projects expanded our knowledge of oceanic and terrestrial deposition processes. Modern deposition science has been significantly influenced by interdisciplinary approaches combining geology, chemistry, physics, and biology. For instance, the study of paleoclimatology relies heavily on analyzing deposited sediments to reconstruct past environmental conditions. Additionally, advances in computational modeling have enabled researchers to simulate complex deposition scenarios, predicting how different factors such as climate change or human activities might impact future deposition patterns. Today, deposition science continues to evolve with ongoing research into topics like biogenic deposition (e.g., coral reefs), chemical precipitation (e.g., mineral deposits), and anthropogenic impacts on natural systems. These studies not only enhance our understanding of Earth's history but also inform strategies for managing resources sustainably and mitigating environmental challenges. Overall, the historical development of deposition science reflects a continuous pursuit of knowledge that has transformed our comprehension of Earth's dynamic surface processes.
Interdisciplinary Nature of Deposition Science
Deposition science is inherently interdisciplinary, drawing upon a wide array of scientific disciplines to understand and manipulate the processes involved in depositing materials. At its core, deposition science integrates principles from physics, chemistry, materials science, and engineering to study how atoms or molecules are deposited onto surfaces. In physics, the study of thermodynamics and kinetics provides insights into the energy states and reaction rates that govern deposition processes. Chemistry plays a crucial role in understanding the chemical reactions and bonding mechanisms that occur during deposition, such as in chemical vapor deposition (CVD) where precursors react to form thin films. Materials science is essential for analyzing the structural and functional properties of the deposited materials, including their mechanical, electrical, and optical characteristics. Engineering disciplines, particularly chemical engineering and mechanical engineering, contribute by designing and optimizing the equipment and processes used for deposition, ensuring scalability and efficiency. The interdisciplinary nature of deposition science also extends to fields like nanotechnology and biotechnology. In nanotechnology, deposition techniques are used to create nanostructured materials with unique properties, such as nanoparticles and nanowires. Biotechnology leverages deposition methods to develop bio-compatible surfaces and coatings for medical devices and tissue engineering applications. Additionally, computational science and simulation play significant roles in modeling deposition processes, predicting outcomes, and optimizing conditions for desired material properties. The collaborative approach in deposition science fosters innovation by combining diverse expertise. For instance, researchers in materials science might work with chemists to develop new precursor molecules for CVD, while engineers design the reactors to control the deposition environment precisely. This synergy not only enhances our understanding of deposition mechanisms but also accelerates the development of new technologies and applications across various industries, from electronics and energy to healthcare and aerospace. In summary, the interdisciplinary nature of deposition science is its strength, allowing for a comprehensive and multifaceted approach to understanding and advancing this field. By integrating knowledge from multiple scientific disciplines, researchers can develop more sophisticated deposition techniques, create novel materials with tailored properties, and drive technological advancements that impact a wide range of sectors.
Processes and Mechanisms in Deposition Science
Deposition science encompasses a broad range of processes and mechanisms that are crucial for understanding how materials form and accumulate in various environments. At its core, deposition science involves the study of how substances are deposited onto surfaces, influencing fields such as materials engineering, environmental science, and biotechnology. This article delves into the multifaceted nature of deposition processes, exploring three key aspects: the types of deposition processes, factors influencing deposition rates and patterns, and techniques for studying these mechanisms. Firstly, we will examine the different types of deposition processes, including chemical, physical, and biological methods. Each type has distinct characteristics and applications, from the precise layering in chemical vapor deposition to the natural accumulation seen in biological systems. Understanding these processes is essential for developing new materials and technologies. Secondly, we will discuss the factors that influence deposition rates and patterns. These factors can include environmental conditions, substrate properties, and the presence of catalysts or inhibitors. Recognizing these influences is vital for optimizing deposition outcomes in various industrial and natural settings. Lastly, we will explore the techniques used to study deposition mechanisms. Advanced methods such as spectroscopy, microscopy, and computational modeling provide insights into the dynamics of deposition at the molecular and atomic levels. These techniques are indispensable for advancing our knowledge of deposition science. By understanding these three critical components—types of deposition processes, influencing factors, and study techniques—we can gain a comprehensive view of how deposition occurs and how it can be controlled and optimized. Let us begin by examining the diverse array of chemical, physical, and biological deposition processes that underpin this fascinating field.
Types of Deposition Processes (Chemical, Physical, Biological)
Deposition processes are fundamental in various scientific and industrial fields, and they can be broadly categorized into chemical, physical, and biological types. **Chemical Deposition** involves the formation of a solid material from a chemical reaction. One common method is Chemical Vapor Deposition (CVD), where gaseous precursors react to form a solid film on a substrate. This process is widely used in semiconductor manufacturing to create thin films of materials like silicon dioxide and silicon nitride. Another example is Electrochemical Deposition (ECD), also known as electroplating, where ions from an electrolyte solution are reduced at an electrode to deposit a metal layer. **Physical Deposition** relies on physical mechanisms rather than chemical reactions. A prominent technique is Physical Vapor Deposition (PVD), which includes methods such as sputtering and evaporation. In sputtering, ions bombard a target material, ejecting atoms that then deposit onto a substrate. Evaporation involves heating a material until it vaporizes and condenses onto a surface. These methods are crucial in the production of thin films for applications like coatings, solar cells, and optical devices. **Biological Deposition**, though less common in industrial contexts, plays a significant role in natural processes and some specialized applications. Biomineralization is a key example where living organisms deposit minerals to form structures like shells, bones, and teeth. This process involves complex interactions between biological molecules and inorganic ions, leading to the formation of highly ordered and functional materials. In biomedical engineering, researchers are exploring biomimetic approaches to replicate these natural deposition processes for creating advanced biomaterials and tissue engineering scaffolds. Understanding these different types of deposition processes is essential for advancing fields such as materials science, nanotechnology, and biotechnology. Each type offers unique advantages and challenges, and selecting the appropriate method depends on the specific requirements of the application, including the desired material properties, substrate compatibility, and environmental considerations. By mastering these deposition techniques, scientists and engineers can develop innovative materials and technologies that drive progress in various industries and improve our daily lives.
Factors Influencing Deposition Rates and Patterns
Deposition rates and patterns are influenced by a complex interplay of factors, each playing a crucial role in the processes and mechanisms of deposition science. **Energy Levels** are a primary factor, as they determine the kinetic energy of particles being deposited. Higher energy levels typically result in faster deposition rates but can also lead to more uniform and dense deposits. **Surface Topography** is another significant influence, with rough surfaces often promoting higher deposition rates due to increased surface area and nucleation sites. The **Chemical Environment**, including the presence of reactants, catalysts, and inhibitors, can significantly alter deposition kinetics. For instance, certain chemicals can enhance or hinder the formation of deposits by altering the reaction pathways. **Temperature** also plays a critical role, as it affects both the kinetic energy of particles and the reaction rates. Generally, higher temperatures increase deposition rates by enhancing particle mobility and reactivity. However, excessive temperatures can lead to undesirable changes in deposit properties or even prevent deposition altogether. **Pressure** is another key factor, particularly in processes like chemical vapor deposition (CVD) where it influences gas-phase reactions and transport mechanisms. Lower pressures often result in more uniform deposits due to reduced gas-phase collisions. The **Substrate Material** is crucial as well, as different materials have varying affinities for the depositing species. Some substrates may catalyze or inhibit deposition through surface reactions, affecting both the rate and pattern of deposition. **Fluid Dynamics**, especially in liquid-phase depositions like electroplating, influence the transport of ions and molecules to the deposition site. Turbulent flow can enhance mass transport but may also introduce irregularities in the deposit pattern. Additionally, **Electromagnetic Fields** can be used to control deposition patterns. For example, in magnetron sputtering, magnetic fields are used to confine and direct plasma ions towards the substrate, resulting in highly uniform thin films. **Time** is a factor that affects the thickness and structure of deposits; longer deposition times generally result in thicker deposits but may also lead to changes in microstructure due to ongoing surface reactions. Understanding these factors is essential for optimizing deposition processes to achieve desired properties and patterns in various applications, from semiconductor manufacturing to biomedical coatings. By carefully controlling these variables, researchers and engineers can tailor the deposition process to produce materials with specific characteristics, thereby advancing the field of deposition science.
Techniques for Studying Deposition Mechanisms
Studying deposition mechanisms involves a range of sophisticated techniques that help scientists understand the complex processes by which materials are deposited onto surfaces. One key method is **Scanning Electron Microscopy (SEM)**, which provides high-resolution images of the deposited material's morphology, allowing researchers to analyze the structure and texture of the deposits. **X-ray Photoelectron Spectroscopy (XPS)** is another crucial technique, enabling the determination of the chemical composition and bonding states of the deposited material, offering insights into the chemical reactions occurring during deposition. **Atomic Force Microscopy (AFM)** is used to study the topography of deposited films at the nanoscale, providing detailed information about surface roughness and grain size. **Transmission Electron Microscopy (TEM)** offers even higher resolution, allowing for the examination of the internal structure and defects within the deposited material. For understanding the kinetic aspects of deposition, **Quartz Crystal Microbalance (QCM)** is employed, measuring changes in mass during the deposition process in real-time. **Spectroscopic Ellipsometry** is a non-destructive technique that measures the optical properties of thin films, helping to determine thickness, refractive index, and extinction coefficient. **Rutherford Backscattering Spectrometry (RBS)** and **Secondary Ion Mass Spectrometry (SIMS)** are used for depth profiling, providing detailed elemental composition as a function of depth within the deposited layer. In addition to these analytical techniques, **Molecular Dynamics Simulations** and **Density Functional Theory (DFT) Calculations** are computational methods that simulate the deposition process at the atomic level, offering insights into the thermodynamic and kinetic factors influencing deposition mechanisms. These simulations can predict the behavior of atoms and molecules during deposition, helping to optimize experimental conditions. **In Situ Monitoring** techniques such as **Infrared Reflection Absorption Spectroscopy (IRRAS)** and **Surface-Enhanced Raman Spectroscopy (SERS)** allow researchers to observe the deposition process in real-time, providing dynamic information about surface reactions and film growth. By combining these experimental and computational techniques, scientists can gain a comprehensive understanding of the complex mechanisms underlying material deposition, which is essential for advancing deposition science and its applications in fields such as electronics, energy storage, and biomedicine.
Applications and Implications of Deposition Science
Deposition science, a multidisciplinary field that encompasses the study of material deposition processes, has far-reaching applications and implications across various domains. This article delves into three critical areas: Environmental Impact and Ecological Significance, Industrial Applications in Materials Science and Engineering, and Role in Geology and Earth Sciences Research. Understanding the environmental impact of deposition processes is crucial as it affects ecosystems and biodiversity, influencing climate change and natural resource management. In the industrial sector, deposition techniques are pivotal in materials science and engineering, enabling the development of advanced materials with tailored properties. Additionally, deposition science plays a vital role in geology and earth sciences research, helping to reconstruct Earth's history and understand geological phenomena. By exploring these facets, we can appreciate the comprehensive impact of deposition science on our environment, technology, and scientific understanding. This article begins by examining the Environmental Impact and Ecological Significance of deposition processes, highlighting their critical role in maintaining ecological balance and mitigating environmental degradation.
Environmental Impact and Ecological Significance
The environmental impact and ecological significance of deposition science are profound and multifaceted. Deposition processes, whether natural or anthropogenic, play a crucial role in shaping ecosystems and influencing environmental health. For instance, sediment deposition in rivers and coastal areas can alter habitats for aquatic species, affecting biodiversity and ecosystem resilience. Similarly, atmospheric deposition of pollutants such as sulfur dioxide and nitrogen oxides contributes to acid rain, which can devastate forests and water bodies by altering pH levels and nutrient cycles. On the other hand, beneficial deposition processes like dust deposition in arid regions can enhance soil fertility and support plant growth, highlighting the complex interplay between deposition and ecological balance. In the context of human activities, understanding deposition science is essential for mitigating environmental impacts. For example, industrial processes that involve the deposition of materials like metals and chemicals must be carefully managed to prevent contamination of soil, water, and air. This is particularly relevant in fields such as mining, where tailings and waste materials can lead to significant environmental degradation if not properly managed. Moreover, deposition science informs strategies for environmental remediation; for instance, using natural or engineered systems to deposit contaminants in less harmful forms or locations. Ecologically, deposition science also underpins conservation efforts. In wetland ecosystems, sediment deposition is critical for maintaining habitat integrity and supporting migratory species. Conversely, excessive sedimentation due to human activities like deforestation or construction can lead to habitat destruction and loss of biodiversity. Understanding these dynamics is vital for developing effective conservation plans that balance human needs with ecological preservation. Furthermore, climate change has amplified the importance of deposition science. Changes in precipitation patterns and increased frequency of extreme weather events alter natural deposition processes, leading to more frequent floods and landslides that reshape landscapes and disrupt ecosystems. Studying these changes helps scientists predict and prepare for future environmental challenges, ensuring more resilient ecosystems and communities. In summary, the environmental impact and ecological significance of deposition science are far-reaching and critical for maintaining healthy ecosystems. By understanding and managing deposition processes effectively, we can mitigate adverse environmental impacts, support biodiversity, and foster sustainable development. This knowledge is indispensable for informing policy, guiding conservation efforts, and ensuring the long-term health of our planet.
Industrial Applications in Materials Science and Engineering
Industrial applications in materials science and engineering are pivotal in transforming theoretical knowledge into practical, innovative solutions that drive technological advancements and economic growth. One of the key areas where this convergence is evident is in the field of deposition science. Deposition science, which involves the controlled placement of material onto a substrate, has far-reaching implications across various industries. In **electronics**, deposition techniques such as chemical vapor deposition (CVD) and physical vapor deposition (PVD) are crucial for fabricating semiconductor devices, thin-film transistors, and other microelectronic components. These methods allow for the precise control of material properties, enabling the creation of high-performance electronic devices that power modern technology. In **aerospace engineering**, advanced materials deposited using techniques like plasma-enhanced CVD (PECVD) or molecular beam epitaxy (MBE) are used to develop lightweight yet incredibly strong composites for aircraft and spacecraft. These materials enhance structural integrity while reducing weight, thereby improving fuel efficiency and overall performance. The **automotive industry** also benefits significantly from deposition science. Coatings deposited via PVD or CVD processes enhance wear resistance and corrosion protection for engine components, gears, and other critical parts. Additionally, these coatings can improve fuel efficiency by reducing friction and enhancing thermal management. In **biomedical engineering**, deposition techniques are used to create biocompatible surfaces for implants and medical devices. For instance, titanium nitride coatings deposited using PVD improve the durability and biocompatibility of orthopedic implants, while thin films of hydroxyapatite deposited via sol-gel methods enhance bone integration. Furthermore, **renewable energy** technologies rely heavily on deposition science. Thin-film solar cells produced using CVD or sputtering techniques offer efficient and cost-effective alternatives to traditional silicon-based solar cells. Similarly, fuel cells and electrolyzers benefit from advanced coatings that enhance catalytic activity and durability. In **construction and architecture**, deposition science contributes to the development of advanced building materials. For example, low-e coatings deposited on glass surfaces using sputtering or CVD methods reduce heat transfer, improving energy efficiency in buildings. Lastly, **consumer goods** such as cookware, tools, and sporting equipment also leverage deposition science. Non-stick coatings like Teflon deposited via PVD or CVD processes make cookware more durable and easier to clean, while wear-resistant coatings on tools extend their lifespan. In summary, the applications of deposition science in materials science and engineering are vast and diverse, driving innovation across multiple industries by enabling the creation of high-performance materials with tailored properties. These advancements not only improve product performance but also contribute to sustainability, energy efficiency, and economic growth.
Role in Geology and Earth Sciences Research
In the realm of geology and earth sciences, deposition science plays a pivotal role in understanding the formation and evolution of Earth's surface. Deposition, the process by which material is deposited or settled, is crucial for reconstructing past environments, climates, and geological events. Geologists rely on deposition science to interpret sedimentary rocks, which are formed through the accumulation of sediments such as sand, silt, and clay. These rocks contain valuable information about ancient ecosystems, including fossil records that provide insights into evolutionary history and biodiversity. Deposition science also informs our understanding of Earth's climate history. Sedimentary deposits like coral reefs, glacial till, and lake sediments serve as archives of past climatic conditions. For instance, ice cores extracted from glaciers contain layers of ice that have been deposited over thousands of years, each layer preserving a snapshot of atmospheric composition and temperature at the time of its formation. Similarly, oceanic sediments like foraminifera shells and deep-sea cores offer detailed records of oceanic conditions and global climate patterns. Furthermore, deposition science has significant implications for natural resource management. Understanding the processes of sediment deposition helps in identifying potential sources of minerals and fossil fuels. For example, oil and gas reservoirs are often found in sedimentary basins where organic-rich sediments have been deposited under specific conditions. Additionally, knowledge of deposition processes aids in predicting and mitigating natural hazards such as landslides, floods, and coastal erosion. In environmental science, deposition science is essential for assessing and managing water quality. Sediments can act as both sinks and sources of pollutants; understanding how sediments are deposited and transported helps in tracing the movement of contaminants through aquatic systems. This knowledge is critical for developing effective strategies to protect water bodies from pollution. Moreover, deposition science contributes to our understanding of geological hazards such as earthquakes and tsunamis. By studying sedimentary layers disturbed by seismic activity or tsunami events, researchers can reconstruct the history of these events and better predict future occurrences. This information is vital for risk assessment and disaster preparedness. In summary, deposition science is a cornerstone of geology and earth sciences research, providing essential insights into Earth's history, climate dynamics, natural resource distribution, environmental health, and geological hazards. Its applications span a wide range of fields from paleoclimatology to resource exploration and environmental management, making it an indispensable tool for advancing our understanding of the Earth's complex systems.