What Does Atp Stand For
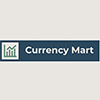
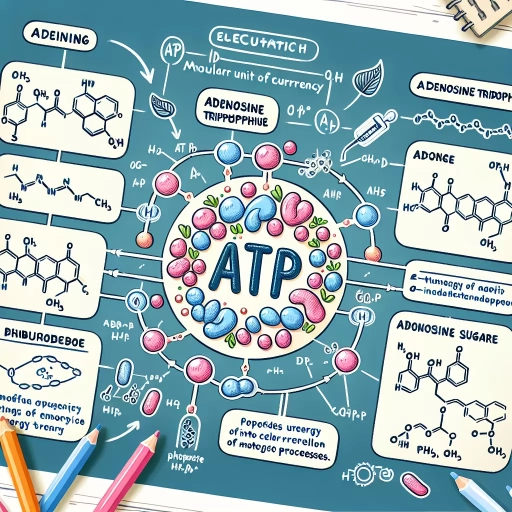
Adenosine triphosphate, commonly abbreviated as ATP, is a molecule that plays a pivotal role in the biological and chemical processes of living organisms. This high-energy molecule is often referred to as the "molecular currency" of energy transfer within cells. To fully appreciate the significance of ATP, it is essential to delve into its fundamental structure and function, which will be explored in the section "Understanding the Basics of ATP." Beyond its basic principles, ATP's influence extends broadly across various biological processes, including metabolism, muscle contraction, and DNA synthesis, as detailed in "The Role of ATP in Biological Processes." Additionally, ATP's importance is not limited to biology; it also has significant implications in diverse scientific fields such as biochemistry, pharmacology, and biotechnology, which will be discussed in "ATP in Various Scientific Fields." By understanding these aspects, we can gain a comprehensive insight into why ATP is so crucial for life. Let us begin by examining the foundational aspects of ATP in "Understanding the Basics of ATP."
Understanding the Basics of ATP
Adenosine triphosphate, or ATP, is the molecular currency of energy transfer within cells, playing a crucial role in various biological processes. To fully grasp the significance of ATP, it is essential to delve into its fundamental aspects. This article will explore the basics of ATP by examining three key areas: **Definition and Full Form**, which clarifies what ATP stands for and its role in cellular metabolism; **Historical Context and Discovery**, which highlights the pivotal moments in the history of ATP's identification and understanding; and **Chemical Structure and Composition**, which details the molecular architecture that enables ATP to function as an energy carrier. By understanding these foundational elements, readers will gain a comprehensive insight into the mechanisms and importance of ATP in biological systems. This knowledge is vital for appreciating how cells harness and utilize energy, making it indispensable for anyone interested in the intricacies of cellular biology. Therefore, let us embark on a journey to **Understanding the Basics of ATP**.
Definition and Full Form
**Understanding the Basics of ATP: Definition and Full Form** Adenosine Triphosphate, commonly abbreviated as ATP, is the molecular currency of energy transfer within cells. The full form of ATP reveals its complex structure and function: Adenosine, a nucleoside composed of a sugar molecule (ribose) and a nitrogenous base (adenine), combined with Triphosphate, indicating three phosphate groups. This intricate molecule plays a pivotal role in cellular metabolism, serving as the primary energy carrier that powers various biological processes. ATP's definition encompasses its role in energy storage and release. When cells need energy, ATP is broken down into Adenosine Diphosphate (ADP) and inorganic phosphate, releasing energy that can be used for cellular activities such as muscle contraction, protein synthesis, and membrane transport. Conversely, during energy-rich states, cells can re-synthesize ATP from ADP and phosphate through processes like cellular respiration and photosynthesis. This dynamic cycle of ATP synthesis and hydrolysis is essential for maintaining cellular homeostasis and supporting life's diverse functions. The full form of ATP highlights its biochemical composition, which includes the adenine base, the ribose sugar, and the triphosphate group. This specific arrangement allows ATP to participate in high-energy phosphate bonds that are easily broken and reformed, making it an efficient energy storage and transfer molecule. Understanding the definition and full form of ATP provides a foundational insight into how cells manage energy, which is crucial for appreciating the broader mechanisms of cellular biology and metabolism. By recognizing ATP's central role in energy transactions, one can better grasp the intricate web of biochemical reactions that sustain life at the cellular level. Thus, delving into the basics of ATP not only clarifies its acronym but also illuminates the fundamental principles governing cellular energy dynamics.
Historical Context and Discovery
The historical context and discovery of ATP (Adenosine Triphosphate) are pivotal in understanding its fundamental role in cellular biology. The journey to uncovering ATP's significance began in the early 20th century, a period marked by rapid advancements in biochemistry. In 1929, German biochemist Karl Lohmann isolated ATP from muscle tissue, although it was initially known as "adenosine triphosphoric acid." This breakthrough was built upon by Sir Alexander Todd, who later elucidated the structure of ATP in the 1940s. However, it was not until the work of Fritz Lipmann in the 1940s that ATP's central role in energy transfer within cells became clear. Lipmann's discovery of the high-energy phosphate bonds in ATP and his introduction of the concept of "energy-rich" compounds revolutionized the field of biochemistry. The subsequent decades saw a flurry of research that further solidified ATP's status as a molecular currency of energy. The work of Peter Mitchell, who proposed the chemiosmotic theory in the 1960s, provided a mechanistic explanation for how ATP is generated during cellular respiration. This theory posits that the energy from the transfer of electrons during oxidative phosphorylation is used to pump protons across cellular membranes, creating a proton gradient that drives the synthesis of ATP. The validation of Mitchell's theory through numerous experiments and observations has made it a cornerstone of modern biochemistry. Understanding the historical context of ATP's discovery highlights the collaborative and iterative nature of scientific progress. From Lohmann's initial isolation to Mitchell's comprehensive theory, each milestone built upon previous findings, gradually revealing the intricate mechanisms by which cells harness and utilize energy. This historical backdrop not only underscores the importance of ATP but also serves as a testament to the power of scientific inquiry and the relentless pursuit of knowledge. As we delve into the basics of ATP, it is crucial to appreciate this rich historical tapestry, which has shaped our current understanding of cellular metabolism and energy production. By recognizing the contributions of these pioneers, we gain a deeper appreciation for the complex biochemical processes that underpin life itself.
Chemical Structure and Composition
Understanding the chemical structure and composition of ATP (Adenosine Triphosphate) is crucial for grasping its role as the primary energy carrier in cells. ATP is a nucleotide composed of three main components: a nitrogenous base called adenine, a sugar molecule known as ribose, and three phosphate groups. The adenine and ribose together form adenosine, which is then linked to the phosphate groups. The phosphate groups are arranged in a linear fashion, with the first phosphate group attached directly to the ribose and the subsequent two forming a chain. This specific arrangement is key to ATP's function. The chemical structure of ATP allows it to store and release energy efficiently. The bonds between the phosphate groups are high-energy bonds, known as phosphoanhydride bonds. When these bonds are hydrolyzed (broken), they release a significant amount of energy, which can be harnessed by the cell to perform various functions such as muscle contraction, protein synthesis, and membrane transport. This process is reversible; ATP can be reformed from ADP (Adenosine Diphosphate) and inorganic phosphate through cellular respiration and other energy-producing pathways. The composition of ATP also includes several functional groups that contribute to its stability and reactivity. The adenine base is a purine, which is one of the two types of nitrogenous bases found in nucleic acids. The ribose sugar provides structural support and serves as a backbone for the molecule. The phosphate groups, aside from their role in energy storage, also contribute to the molecule's solubility and ability to interact with enzymes. In cellular metabolism, the structure and composition of ATP enable it to act as a versatile energy currency. Enzymes such as kinases and phosphatases can easily recognize and interact with ATP due to its specific chemical configuration. This specificity ensures that energy is transferred efficiently and accurately within the cell. Moreover, the chemical properties of ATP allow it to be synthesized and broken down rapidly, making it an ideal molecule for immediate energy needs. In summary, the chemical structure and composition of ATP are finely tuned for its role as a cellular energy carrier. The arrangement of adenine, ribose, and phosphate groups in ATP allows for the efficient storage and release of energy, making it indispensable for various cellular processes. Understanding these details provides a solid foundation for appreciating how ATP functions within the broader context of cellular metabolism and energy production.
The Role of ATP in Biological Processes
Adenosine triphosphate (ATP) is the molecular currency of energy in biological systems, playing a pivotal role in various cellular processes that sustain life. This essential molecule is at the heart of energy transfer and metabolism, facilitating the conversion of chemical energy into usable forms that power cellular activities. Beyond its role in metabolism, ATP is crucial for cellular functions and signaling, enabling cells to communicate and respond to their environment. Additionally, ATP is indispensable for muscle contraction and movement, providing the energy necessary for muscle fibers to contract and relax. Understanding the multifaceted role of ATP in these biological processes is fundamental to grasping how cells function and how life is sustained. In this article, we will delve into the specifics of ATP's involvement in energy transfer and metabolism, its significance in cellular functions and signaling, and its importance in muscle contraction and movement. By exploring these aspects, we will gain a deeper understanding of the basics of ATP and its central position in biological processes.
Energy Transfer and Metabolism
Energy transfer and metabolism are fundamental processes that underpin the vitality of all living organisms. At the heart of these processes is the molecule adenosine triphosphate (ATP), which serves as the primary energy currency of the cell. Metabolism, a complex network of biochemical reactions, is divided into two main categories: catabolism and anabolism. Catabolic pathways break down complex molecules such as carbohydrates, fats, and proteins into simpler forms, releasing energy that is captured in the form of ATP. Conversely, anabolic pathways use this stored energy to synthesize new molecules necessary for growth, repair, and maintenance of cellular structures. The transfer of energy from catabolic processes to ATP is highly efficient due to the cellular machinery known as the electron transport chain (ETC) located in the mitochondria. Here, electrons from high-energy molecules are passed through a series of protein complexes, generating a proton gradient across the mitochondrial membrane. This gradient drives the production of ATP through the process of chemiosmosis, where protons flow back across the membrane, driving the enzyme ATP synthase to produce ATP from adenosine diphosphate (ADP) and inorganic phosphate. In addition to its role in energy storage and transfer, ATP plays a crucial role in various cellular functions. It powers muscle contraction by binding to myosin heads, enabling them to move along actin filaments. ATP also fuels active transport mechanisms that maintain cellular homeostasis by pumping ions and molecules against concentration gradients. Furthermore, ATP is essential for DNA replication and repair, as well as protein synthesis, where it provides the energy needed for peptide bond formation. The dynamic balance between ATP production and consumption ensures that cells maintain optimal energy levels. This balance is tightly regulated by feedback mechanisms that adjust metabolic rates according to cellular needs. For instance, when ATP levels are high, signaling pathways can inhibit catabolic processes to prevent excessive energy production. Conversely, low ATP levels trigger the activation of catabolic pathways to increase energy yield. In summary, energy transfer and metabolism are intricately linked processes that rely on ATP as their central axis. The efficient production and utilization of ATP enable cells to perform a wide range of functions essential for life, from basic metabolic processes to complex cellular activities. Understanding these mechanisms provides profound insights into how living organisms sustain their vitality and adapt to changing environments.
Cellular Functions and Signaling
Cellular functions and signaling are intricately linked processes that underpin the complex operations within living cells. At the heart of these processes is the molecule ATP (adenosine triphosphate), which serves as a universal energy currency. Cellular signaling, a critical aspect of cellular function, involves the transmission of information from one part of the cell to another or between cells. This communication is essential for coordinating activities such as growth, differentiation, and response to environmental changes. ATP plays a pivotal role in cellular signaling pathways by providing the necessary energy for various signaling molecules to perform their functions. For instance, in the context of protein kinases—enzymes that add phosphate groups to other proteins—ATP is used as a phosphate donor. This phosphorylation event can activate or inhibit target proteins, thereby modulating downstream signaling cascades. Additionally, ATP is required for the functioning of ion pumps and channels that regulate the flow of ions across cell membranes, influencing electrical signals and maintaining cellular homeostasis. Another key aspect of cellular signaling is the involvement of second messengers like cyclic AMP (cAMP) and calcium ions. These molecules are generated through ATP-dependent reactions and serve as intermediaries in signal transduction pathways. For example, cAMP is synthesized from ATP by the enzyme adenylate cyclase and acts to activate protein kinase A (PKA), which then phosphorylates various target proteins to elicit specific cellular responses. Moreover, ATP is crucial for the maintenance of cellular structure and function through its role in cytoskeletal dynamics. The cytoskeleton, composed of microtubules, microfilaments, and intermediate filaments, requires ATP for polymerization and depolymerization processes that enable cell shape changes, division, and movement. This dynamic reorganization is vital for processes such as muscle contraction, where myosin heads use ATP to slide along actin filaments. In summary, ATP is indispensable for both cellular functions and signaling mechanisms. Its energy is harnessed to drive enzymatic reactions, ion transport, and cytoskeletal rearrangements that are fundamental to maintaining cellular integrity and facilitating communication within and between cells. The multifaceted role of ATP underscores its importance as a central molecule in biological processes, ensuring that cells can respond appropriately to internal and external stimuli while sustaining their basic metabolic activities.
Importance in Muscle Contraction and Movement
Muscle contraction and movement are fundamental biological processes that underpin the very essence of life, from the simplest reflexes to complex motor functions. At the heart of these processes lies the critical role of ATP (Adenosine Triphosphate), which serves as the primary energy currency of the cell. When muscles contract, they require a rapid and efficient source of energy to fuel the sliding filament mechanism that drives muscle shortening. Here, ATP plays an indispensable role by providing the necessary energy through its hydrolysis to ADP (Adenosine Diphosphate) and inorganic phosphate. The process begins with the binding of actin and myosin filaments in muscle fibers, a step that is energetically unfavorable without the input of ATP. As ATP binds to the myosin head, it undergoes hydrolysis, releasing energy that powers the conformational change in the myosin head. This change allows the myosin head to pull the actin filament along its length, resulting in muscle contraction. The speed and force of this contraction are directly dependent on the availability of ATP; without it, muscles would be unable to generate the necessary force for movement. Moreover, ATP's role extends beyond just initiating contraction; it is also crucial for muscle relaxation. During relaxation, ATP is used to pump calcium ions back into the sarcoplasmic reticulum, reducing intracellular calcium levels and allowing the muscle to return to its resting state. This dynamic interplay between contraction and relaxation, both fueled by ATP, enables muscles to perform a wide range of functions from maintaining posture to executing precise movements. In addition to its direct involvement in muscle mechanics, ATP supports other ancillary processes that are vital for efficient muscle function. For instance, ATP is used in the synthesis of creatine phosphate, a high-energy compound that rapidly replenishes ATP stores during intense muscle activity. This buffering system ensures that muscles can sustain activity over longer periods without significant fatigue. In summary, the importance of muscle contraction and movement cannot be overstated, and ATP is central to these processes. Its ability to rapidly provide energy makes it an essential component of both the initiation and regulation of muscle activity. Without ATP, the intricate dance of actin and myosin filaments would grind to a halt, rendering movement impossible. Thus, understanding the role of ATP in muscle contraction highlights its broader significance in biological processes, underscoring why it is often referred to as the "molecular unit of currency" for energy transfer within cells.
ATP in Various Scientific Fields
Adenosine triphosphate (ATP) is a molecule that plays a pivotal role across various scientific fields, serving as the primary energy currency of the cell. Its significance extends far beyond its biochemical function, influencing multiple disciplines in profound ways. In biochemistry and molecular biology, ATP is crucial for understanding metabolic pathways and cellular processes, driving reactions that sustain life. In neuroscience, ATP's role in brain function is multifaceted, contributing to neurotransmission and synaptic plasticity, which are fundamental to cognitive processes and behavior. Additionally, in medical research and treatment, ATP's implications are vast, from understanding disease mechanisms to developing therapeutic strategies. This article delves into these diverse applications, highlighting how ATP's multifunctional nature makes it an indispensable component of scientific inquiry. By exploring its roles in biochemistry, neuroscience, and medical research, we gain a deeper appreciation for the molecule's importance. To fully grasp these complex applications, it is essential to first understand the basics of ATP. **Understanding the Basics of ATP**
Applications in Biochemistry and Molecular Biology
In the realm of biochemistry and molecular biology, ATP (adenosine triphosphate) plays a pivotal role, serving as the primary energy currency of cells. Its applications are multifaceted and indispensable across various scientific fields. In biochemistry, ATP is crucial for understanding metabolic pathways, such as glycolysis, the citric acid cycle, and oxidative phosphorylation, where it acts as both an energy donor and acceptor. This molecule facilitates the transfer of energy from one reaction to another, enabling cells to perform a wide range of functions including muscle contraction, protein synthesis, and membrane transport. For instance, in muscle cells, ATP hydrolysis powers the sliding filament mechanism that underlies muscle contraction, while in neurons, ATP is essential for maintaining the resting membrane potential and facilitating neurotransmitter release. In molecular biology, ATP's significance extends to DNA replication and repair processes. During DNA replication, ATP is required for the unwinding of double-stranded DNA by helicases and for the synthesis of new DNA strands by DNA polymerases. Additionally, ATP-dependent enzymes like topoisomerases help manage DNA supercoiling and tangles, ensuring proper chromosome segregation during cell division. In DNA repair mechanisms such as mismatch repair and nucleotide excision repair, ATP is necessary for the activation of repair enzymes that correct genetic errors. Furthermore, ATP's role in signal transduction pathways is critical for cellular communication and response to environmental stimuli. For example, ATP can act as a signaling molecule itself when released into the extracellular environment, binding to purinergic receptors on nearby cells to initiate various physiological responses. This extracellular ATP signaling is involved in processes such as inflammation, immune response, and even neurotransmission. The study of ATP in biochemistry and molecular biology also has significant implications for medical research and drug development. Understanding how ATP is produced and utilized in different disease states can lead to the development of targeted therapies. For instance, drugs that modulate ATP production or consumption are being explored for treating conditions like cancer, where altered energy metabolism is a hallmark of tumor cells. In summary, ATP's central role in biochemistry and molecular biology underscores its importance as a fundamental molecule in cellular processes. Its involvement in energy metabolism, DNA transactions, signal transduction, and disease pathology makes it a focal point of research across these scientific disciplines. As our understanding of ATP's functions continues to evolve, so too do the potential applications in fields ranging from basic research to clinical medicine.
Role in Neuroscience and Brain Function
In the realm of neuroscience, ATP (adenosine triphosphate) plays a pivotal role in maintaining the intricate functions of the brain. As the primary energy currency of cells, ATP is essential for neuronal activity and synaptic transmission. Within neurons, ATP is generated through cellular respiration, primarily in the mitochondria, and is then transported to various cellular compartments where it fuels critical processes. For instance, ATP is crucial for the operation of ion pumps that regulate the electrical properties of neurons, such as the sodium-potassium pump, which helps maintain the resting membrane potential and facilitates action potentials. This energy is also vital for neurotransmitter release and reuptake at synapses, enabling communication between neurons and forming the basis of cognitive functions like learning and memory. Moreover, ATP's role extends to neuroprotection and neuroplasticity. It supports the function of various ATP-dependent enzymes involved in DNA repair, protein folding, and degradation pathways, which are essential for neuronal survival and resilience against oxidative stress and other forms of cellular damage. Additionally, ATP influences synaptic plasticity by powering the machinery necessary for long-term potentiation (LTP) and long-term depression (LTD), mechanisms that underpin learning and memory consolidation. The significance of ATP in brain function is further underscored by its involvement in neurodegenerative diseases. Conditions such as Alzheimer's disease, Parkinson's disease, and Huntington's disease are characterized by mitochondrial dysfunction and impaired ATP production, leading to neuronal energy deficits that contribute to disease progression. Understanding the role of ATP in these contexts has opened avenues for therapeutic interventions aimed at enhancing mitochondrial function and improving energy metabolism in the brain. In summary, ATP's multifaceted role in neuroscience underscores its importance as a fundamental component of brain function. From powering neuronal activity to supporting neuroprotection and plasticity, ATP is indispensable for maintaining the complex operations of the brain. Its dysfunction can have profound implications for neurological health, making it a critical area of study in both basic neuroscience research and clinical neurology.
Implications in Medical Research and Treatment
In the realm of medical research and treatment, the implications of ATP (Adenosine Triphosphate) are profound and multifaceted. ATP, often referred to as the "molecular currency" of energy transfer, plays a crucial role in cellular metabolism and function. Its significance extends beyond basic cellular processes to influence various aspects of medical science. Firstly, understanding ATP's role in cellular respiration is essential for developing treatments for metabolic disorders. For instance, mitochondrial diseases, which affect the mitochondria's ability to produce ATP, can lead to severe health issues such as muscular dystrophy and neurological disorders. Research into these conditions often focuses on enhancing mitochondrial function or finding alternative pathways for energy production, thereby improving patient outcomes. Moreover, ATP's involvement in signaling pathways makes it a key player in immune response and inflammation. ATP can act as an extracellular signaling molecule, influencing immune cell activation and cytokine release. This knowledge has led to the development of therapeutic strategies targeting purinergic receptors, which are involved in ATP signaling, to manage conditions like arthritis and autoimmune diseases. In cancer research, ATP's role is equally significant. Cancer cells often exhibit altered metabolism, known as the Warburg effect, where they preferentially use glycolysis for energy production even in the presence of oxygen. Understanding these metabolic shifts can help in designing targeted therapies that exploit the unique energy requirements of cancer cells, potentially leading to more effective treatments with fewer side effects. Additionally, ATP's function in neurotransmission and synaptic plasticity makes it relevant to neurological disorders such as Alzheimer's disease and Parkinson's disease. Research into ATP's role in maintaining neuronal health and function could pave the way for novel therapeutic approaches aimed at preserving cognitive function and motor control. Finally, advancements in gene therapy and regenerative medicine also rely on a deep understanding of ATP's cellular mechanisms. For example, gene therapies aimed at correcting genetic defects that impair ATP production can offer hope for treating inherited metabolic disorders. Similarly, regenerative therapies that enhance cellular energy metabolism could improve tissue repair and organ function. In summary, the implications of ATP in medical research and treatment are vast and varied. From metabolic disorders to cancer, immune diseases, neurological conditions, and regenerative medicine, understanding ATP's multifaceted roles continues to drive innovation and improvement in patient care. As research continues to unravel the complexities of ATP's function, it holds the promise of unlocking new therapeutic avenues that could transform the landscape of medical treatment.