What Is Particle Theory
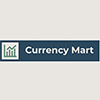
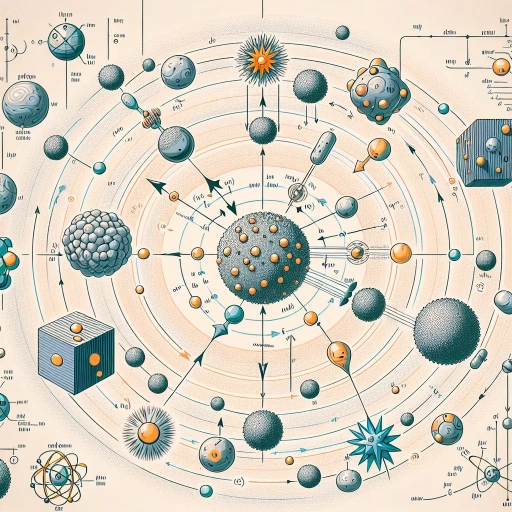
Particle theory, a foundational concept in physics, underpins our understanding of the microscopic world. This theory posits that matter is composed of tiny, indivisible particles rather than being continuous. At its core, particle theory explains how these particles interact and behave, forming the basis of modern physics. The article delves into three key aspects of particle theory: **Introduction to Particle Theory**, which explores the historical development and fundamental principles of the concept; **Core Components of Particle Theory**, which examines the essential elements such as particles, forces, and interactions; and **Applications and Implications of Particle Theory**, which discusses how this theory influences various fields from chemistry to engineering. By understanding these components, we gain insight into the intricate mechanisms governing our universe. Let us begin by diving into the **Introduction to Particle Theory**, where we will uncover the origins and basic tenets of this pivotal scientific framework.
Introduction to Particle Theory
Particle theory, a cornerstone of modern physics, has evolved significantly over centuries, transforming our understanding of the fundamental nature of matter and energy. This article delves into the historical development of particle theory, tracing its roots from ancient philosophers to the groundbreaking discoveries of the 20th century. We will explore key concepts and principles that underpin this theory, including the behavior of particles at the atomic and subatomic levels, quantum mechanics, and the role of forces such as electromagnetism and the strong and weak nuclear forces. Additionally, we will discuss the importance of particle theory in modern science, highlighting its applications in fields like particle physics, materials science, and medical research. By examining these aspects, this introduction to particle theory aims to provide a comprehensive overview of how this field has shaped our current scientific landscape and continues to drive innovation and discovery. Transitioning seamlessly into the heart of the matter, let us begin our journey with an **Introduction to Particle Theory**.
Historical Development of Particle Theory
The historical development of particle theory is a rich and intricate narrative that spans centuries, reflecting the evolving understanding of the fundamental nature of matter and energy. The earliest seeds of particle theory were sown in ancient Greece, where philosophers such as Democritus proposed the existence of indivisible particles called atoms around 450 BCE. This concept lay dormant for centuries until the 17th and 18th centuries, when scientists like Pierre Gassendi and Antoine Lavoisier revived atomic theories. However, it was John Dalton in the early 19th century who formulated the modern atomic theory, positing that elements are composed of small, indivisible particles called atoms and that compounds are formed when atoms of different elements combine in whole number ratios. The late 19th and early 20th centuries saw a significant leap forward with the discovery of subatomic particles. J.J. Thomson's discovery of the electron in 1897 revealed that atoms were not indivisible but rather composed of smaller particles. This was followed by Ernest Rutherford's famous gold foil experiment in 1909, which led to the development of the nuclear model of the atom, where a small nucleus containing protons is surrounded by electrons. The discovery of the neutron by James Chadwick in 1932 completed the basic structure of the atom as we understand it today. The mid-20th century ushered in the era of particle physics with the advent of high-energy accelerators and detectors. This period saw the discovery of a plethora of subatomic particles, including mesons, baryons, and leptons, which were initially thought to be fundamental but later found to be composed of even smaller entities known as quarks and gluons. The development of the Standard Model of particle physics in the 1970s, largely through the work of physicists such as Murray Gell-Mann and George Zweig, provided a comprehensive framework for understanding these particles and their interactions. In recent decades, advancements in experimental techniques and theoretical models have continued to refine our understanding. The discovery of the Higgs boson in 2012 at CERN's Large Hadron Collider confirmed a key prediction of the Standard Model and marked a major milestone in particle physics. Ongoing research aims to address remaining questions, such as the nature of dark matter and dark energy, which are believed to make up a significant portion of the universe but remain poorly understood. The historical development of particle theory is thus a story of continuous discovery and refinement, reflecting humanity's relentless pursuit to understand the fundamental building blocks of our universe.
Key Concepts and Principles
In the realm of particle theory, several key concepts and principles form the foundational framework that underpins our understanding of the microscopic world. At its core, particle theory posits that matter is composed of discrete particles rather than being continuous. This idea is central to both classical and quantum mechanics. One of the primary principles is the concept of **wave-particle duality**, which suggests that particles, such as electrons and photons, can exhibit both wave-like and particle-like behavior depending on how they are observed. This duality is a cornerstone of quantum mechanics and has been experimentally verified through various studies, including the famous double-slit experiment. Another crucial concept is **quantization**, which states that certain physical properties, like energy and angular momentum, come in discrete packets (quanta) rather than being continuous. This principle is fundamental to understanding phenomena such as the quantized energy levels in atoms and molecules. The **Heisenberg Uncertainty Principle** is another pivotal concept, asserting that it is impossible to know both the position and momentum of a particle with infinite precision simultaneously. This principle highlights the inherent probabilistic nature of quantum mechanics. The **Standard Model of particle physics** provides a comprehensive framework for understanding the behavior of fundamental particles and forces. It categorizes particles into two main types: **fermions** (matter particles like quarks and leptons) and **bosons** (force-carrying particles like photons and gluons). The Standard Model also explains how these particles interact via fundamental forces such as electromagnetism, the strong nuclear force, and the weak nuclear force. Additionally, **symmetry principles** play a significant role in particle theory. Symmetries such as rotational symmetry, translational symmetry, and gauge symmetries help predict the behavior of particles under different conditions. For instance, the conservation laws derived from these symmetries (like conservation of energy and momentum) are essential in predicting outcomes in particle interactions. Finally, **relativity**—both special and general—integrates seamlessly with particle theory by providing a framework for understanding how particles behave at high speeds and in strong gravitational fields. The relativistic mass-energy equivalence (\(E = mc^2\)) is a direct consequence of special relativity and has profound implications for particle physics. These concepts and principles collectively form a robust theoretical structure that allows scientists to predict and explain a wide range of phenomena observed in experiments involving subatomic particles. By understanding these foundational ideas, researchers can delve deeper into the mysteries of the microscopic universe, driving advancements in fields ranging from materials science to cosmology.
Importance in Modern Science
In the realm of modern science, particle theory stands as a cornerstone of our understanding of the universe. This theory, which posits that matter and energy are composed of discrete particles rather than being continuous, has revolutionized various fields including physics, chemistry, and materials science. The importance of particle theory lies in its ability to explain phenomena at the smallest scales, from the behavior of electrons in atoms to the interactions of subatomic particles in high-energy collisions. At its core, particle theory provides a framework for understanding the fundamental building blocks of matter. The discovery of particles such as electrons, protons, neutrons, and later quarks and leptons, has allowed scientists to construct models that predict and explain a wide range of physical phenomena. For instance, the Standard Model of particle physics, which is a culmination of decades of research and experimentation, describes how these particles interact through fundamental forces like electromagnetism and the strong and weak nuclear forces. The implications of particle theory extend far beyond theoretical physics. In chemistry, understanding the behavior of electrons and atomic nuclei is crucial for predicting chemical reactions and properties of materials. This knowledge has led to significant advancements in fields such as materials science and nanotechnology, where researchers design new materials with tailored properties by manipulating particles at the atomic level. Moreover, particle theory has practical applications in technology and medicine. For example, particle accelerators used in high-energy physics research also find applications in cancer treatment through proton therapy. Here, precise beams of protons are used to target and destroy cancer cells while minimizing damage to surrounding healthy tissue. Furthermore, advancements in particle theory have driven innovation in computing and electronics. The development of transistors, which are fundamental components of modern electronics, relies on an understanding of how electrons behave in semiconductors—a direct application of particle theory. In conclusion, the importance of particle theory in modern science cannot be overstated. It underpins our current understanding of the universe from the smallest subatomic particles to complex systems like stars and galaxies. As research continues to push the boundaries of what we know about these particles and their interactions, we can expect further breakthroughs that will shape future technologies and deepen our comprehension of the cosmos. Thus, an introduction to particle theory is not just an academic exercise but a gateway to understanding some of the most profound and impactful ideas in contemporary science.
Core Components of Particle Theory
Particle theory, a cornerstone of modern physics, delves into the fundamental building blocks of matter and the interactions that govern their behavior. At its core, this theory is composed of several key components that collectively explain the structure and dynamics of the physical world. First, understanding **Atoms and Molecules** is crucial, as these are the basic units of chemical substances and the foundation upon which more complex structures are built. Next, the **Subatomic Particles: Protons, Neutrons, and Electrons** play a pivotal role in defining the properties of atoms and molecules, each contributing uniquely to the overall characteristics of matter. Finally, **Interactions and Forces Between Particles** are essential for explaining how these subatomic entities interact with each other, shaping the behavior of matter at various scales. By exploring these core components, we gain a profound insight into the nature of reality itself. This article will delve into each of these aspects in detail, providing a comprehensive introduction to particle theory that will illuminate the intricate mechanisms underlying our universe. Transitioning seamlessly from these foundational elements, we will embark on an **Introduction to Particle Theory**, where the intricate dance of particles and forces is revealed in all its complexity.
Atoms and Molecules
Atoms and molecules are the fundamental building blocks of matter, and understanding their structure and behavior is crucial for grasping the core components of particle theory. Atoms, the smallest units of a chemical element, consist of three primary subatomic particles: protons, neutrons, and electrons. Protons and neutrons reside in the nucleus at the atom's center, while electrons orbit around it in electron shells. The number of protons in an atom determines its chemical identity, forming the basis for the periodic table. Neutrons contribute to the atom's mass but do not affect its charge. Molecules, on the other hand, are formed when two or more atoms share or exchange electrons to achieve a stable electronic configuration. This bonding process can result in covalent, ionic, or metallic bonds, each with distinct properties. Covalent bonds involve the sharing of electron pairs between atoms, typically found in molecules like water (H₂O) and carbon dioxide (CO₂). Ionic bonds are formed through the transfer of electrons between atoms, leading to the formation of ions that are electrostatically attracted to each other, as seen in compounds like sodium chloride (NaCl). Metallic bonds involve a "sea" of electrons surrounding a lattice of metal ions, characteristic of metals like copper and iron. The interactions between atoms and molecules govern the physical and chemical properties of substances. For instance, the arrangement of electrons in an atom influences its reactivity and ability to form bonds. The shape and polarity of molecules determine their solubility, boiling point, and other physical properties. Understanding these interactions is essential for predicting how substances will behave under different conditions, which is a cornerstone of particle theory. Moreover, the study of atoms and molecules has led to significant advancements in various fields. In chemistry, it has enabled the synthesis of new materials with tailored properties. In physics, it has contributed to our understanding of quantum mechanics and the behavior of matter at the atomic and subatomic level. In biology, it underpins our knowledge of biochemical processes and the structure of biomolecules such as DNA and proteins. In summary, atoms and molecules are not just abstract concepts but tangible entities that form the basis of all matter. Their structure, interactions, and bonding mechanisms are fundamental to particle theory, allowing us to explain a wide range of phenomena from the simplest chemical reactions to complex biological processes. By delving into the intricacies of these microscopic worlds, we gain a deeper appreciation for the intricate dance of particles that constitutes our universe.
Subatomic Particles: Protons, Neutrons, and Electrons
At the heart of particle theory lies the understanding of subatomic particles, which are the fundamental building blocks of matter. These particles include protons, neutrons, and electrons, each playing a crucial role in the structure and properties of atoms. **Protons** are positively charged particles that reside in the nucleus of an atom, contributing to its overall positive charge. The number of protons in an atom determines the element to which it belongs, with each element having a unique number of protons known as its atomic number. **Neutrons**, on the other hand, are neutral particles that also reside in the nucleus alongside protons. They contribute to the mass of the atom but do not affect its charge. The combination of protons and neutrons in the nucleus gives rise to different isotopes of an element, which are atoms of the same element with varying numbers of neutrons. **Electrons** are negatively charged particles that orbit around the nucleus in electron shells or energy levels. The number of electrons in a neutral atom is equal to the number of protons, and this balance is essential for maintaining the stability of the atom. Electrons are arranged in specific energy levels or shells around the nucleus, and their arrangement determines the chemical properties of an element. Understanding these subatomic particles is pivotal for grasping various phenomena in physics and chemistry. For instance, chemical reactions involve the interaction and rearrangement of electrons between atoms, while nuclear reactions involve changes to the protons and neutrons within the nucleus. The study of these particles has led to significant advancements in fields such as nuclear physics, materials science, and quantum mechanics. In nuclear physics, the behavior of protons and neutrons helps explain phenomena like nuclear fission and fusion, which are critical for energy production and understanding stellar processes. In materials science, the arrangement of electrons in solids influences their electrical and thermal properties, making it possible to design materials with specific characteristics. Quantum mechanics, a fundamental theory in physics, provides a detailed framework for understanding the behavior of electrons and other subatomic particles at the atomic and subatomic level. The discovery and study of these particles have been milestones in scientific history. The discovery of the electron by J.J. Thomson in 1897 marked the beginning of subatomic particle research. Later, Ernest Rutherford's famous gold foil experiment led to the discovery of the nucleus and subsequently the proton. Neutrons were discovered by James Chadwick in 1932, completing our basic understanding of atomic structure. These findings have not only expanded our knowledge of matter but have also paved the way for technological innovations and further scientific inquiry into the nature of reality itself. In summary, protons, neutrons, and electrons are the core components that underpin particle theory, enabling us to understand the intricate structure and behavior of atoms. Their interactions and arrangements form the basis of chemical and physical phenomena, driving advancements in various scientific disciplines and technological applications. As research continues to delve deeper into the properties and behaviors of these subatomic particles, we gain a more profound appreciation for the intricate complexity and beauty of the atomic world.
Interactions and Forces Between Particles
Interactions and forces between particles are fundamental aspects of particle theory, governing the behavior and dynamics of the microscopic world. At the heart of these interactions lie the four fundamental forces of nature: gravity, electromagnetism, the strong nuclear force, and the weak nuclear force. Each force plays a distinct role in how particles interact with one another. **Gravity**, the weakest but most pervasive force, acts between all particles with mass or energy. While it is negligible at the particle level compared to other forces, it becomes significant at larger scales, shaping the cosmos as we know it. **Electromagnetism**, mediated by photons, is responsible for interactions between charged particles. This force underpins a wide range of phenomena, from chemical bonding to electromagnetic waves. The **strong nuclear force**, mediated by gluons, is the strongest force and holds quarks together within protons and neutrons, and these nucleons within atomic nuclei. It is crucial for the stability of matter itself. The **weak nuclear force**, mediated by W and Z bosons, is involved in certain types of radioactive decay and plays a key role in processes such as beta decay, where a neutron transforms into a proton. These forces are not just abstract concepts but are experimentally verified through various particle physics experiments. For instance, the existence of gluons was confirmed through deep inelastic scattering experiments, while the discovery of W and Z bosons at CERN in the 1980s provided direct evidence for the weak force. Understanding these interactions is crucial for advancing our knowledge of particle theory. For example, quantum field theory (QFT) provides a framework to describe how particles interact via these forces. QFT predicts the existence of virtual particles that mediate these interactions and has been incredibly successful in predicting experimental outcomes. Moreover, the study of particle interactions has led to significant technological advancements. Particle accelerators, which accelerate particles to nearly the speed of light to study their collisions, have driven innovations in materials science, medical imaging, and computing. The Large Hadron Collider (LHC), for instance, has not only confirmed the existence of the Higgs boson but also pushed the boundaries of engineering and computational science. In summary, the interactions and forces between particles form the backbone of particle theory. These forces dictate how particles behave, interact, and form the building blocks of matter. By understanding these interactions, scientists can unravel the mysteries of the universe at its most fundamental level, leading to profound insights into the nature of reality itself. This understanding is not merely theoretical; it has practical implications that drive technological innovation and our broader understanding of the cosmos.
Applications and Implications of Particle Theory
Particle theory, a cornerstone of modern physics, has far-reaching implications that span multiple disciplines. This theory, which posits that matter is composed of tiny, indivisible particles such as atoms and subatomic particles, underpins our understanding of various phenomena. In this article, we will delve into the applications and implications of particle theory across three critical areas. First, we will explore how particle theory influences **Chemical Reactions and Bonding**, shedding light on the fundamental interactions that govern the formation and breaking of chemical bonds. Next, we will examine the **Physical Properties of Materials**, highlighting how the behavior of particles at the atomic and subatomic level determines the characteristics of different materials. Finally, we will discuss **Technological Innovations and Future Directions**, showcasing how advancements in particle theory have driven technological breakthroughs and what future developments might hold. By understanding these applications, we can appreciate the profound impact of particle theory on our daily lives and the scientific landscape. This journey into the applications and implications of particle theory will set the stage for a deeper exploration of its foundational principles in the **Introduction to Particle Theory**.
Chemical Reactions and Bonding
Chemical reactions and bonding are fundamental concepts that underpin the applications and implications of particle theory. At the heart of these processes lies the interaction between atoms, which are composed of particles such as electrons, protons, and neutrons. According to particle theory, atoms are not indivisible but rather consist of these subatomic particles. This understanding is crucial for explaining how chemical bonds form and how chemical reactions occur. In chemical bonding, atoms share or exchange electrons to achieve a stable electronic configuration, often mimicking the noble gas configuration. This sharing or exchange results in the formation of covalent, ionic, or metallic bonds. For instance, in covalent bonding, atoms share pairs of electrons to form molecules like water (H₂O) or methane (CH₄). In ionic bonding, electrons are transferred between atoms, leading to the formation of ions that are electrostatically attracted to each other, as seen in sodium chloride (NaCl). The nature of these bonds is directly influenced by the arrangement and behavior of electrons around the nucleus, a principle rooted in particle theory. Chemical reactions involve the breaking and forming of these chemical bonds. Particle theory helps explain the mechanisms behind these reactions by detailing how electrons are rearranged during the process. For example, in a combustion reaction like the burning of methane, the carbon-hydrogen bonds in methane are broken, and new bonds are formed between carbon and oxygen to produce carbon dioxide and water. This process is driven by the energy changes associated with the formation and breaking of bonds, which can be understood through the principles of thermodynamics and kinetics, both of which rely on particle theory. The implications of these concepts are far-reaching. In fields such as materials science, understanding chemical bonding allows for the design and synthesis of new materials with specific properties. For instance, polymers like polyethylene are created through the formation of long chains of covalently bonded carbon and hydrogen atoms. In pharmaceuticals, the precise manipulation of chemical reactions and bonding is critical for synthesizing drugs that target specific biological pathways. Additionally, in environmental science, knowledge of chemical reactions helps in understanding and mitigating pollution by identifying how pollutants interact with other substances in the environment. In summary, the principles of particle theory provide a foundational framework for understanding chemical reactions and bonding. By elucidating the behavior of subatomic particles, particle theory enables us to predict and manipulate chemical processes, leading to significant advancements across various scientific disciplines. This deep understanding not only enhances our ability to develop new technologies but also informs our approach to solving complex environmental and health challenges.
Physical Properties of Materials
The physical properties of materials are fundamentally influenced by the principles of particle theory, which posits that matter is composed of discrete particles such as atoms and molecules. Understanding these properties is crucial for a wide range of applications and implications. **Density**, for instance, is a key physical property that arises from the arrangement and mass of particles within a material. Materials with tightly packed particles tend to have higher densities, while those with more space between particles have lower densities. This property is vital in engineering, where the selection of materials for structural integrity or buoyancy depends on their density. **Thermal Conductivity** is another significant property that is directly related to the movement and interaction of particles. In metals, free electrons can move freely, carrying heat energy efficiently, making them good conductors. In contrast, insulators have particles that are tightly bound, limiting the transfer of heat energy. This distinction is critical in fields like electronics and construction, where materials must be chosen based on their ability to conduct or insulate heat. **Melting and Boiling Points** are also determined by the intermolecular forces between particles. Materials with strong intermolecular forces require more energy to overcome these forces, resulting in higher melting and boiling points. This property is essential in chemistry and materials science, where it influences the synthesis and processing of materials. The **Elasticity** of a material, or its ability to return to its original shape after deformation, is a function of the bonding between particles. Materials with strong covalent bonds tend to be more elastic, while those with weaker bonds may deform permanently. This property is crucial in mechanical engineering, where the resilience of materials under stress is a key consideration. Furthermore, the **Optical Properties** of materials, such as transparency or reflectivity, are influenced by how particles interact with light. In transparent materials like glass, particles allow light to pass through without significant scattering, while in opaque materials like metals, particles absorb or reflect light. These properties are vital in optics and photonics, where materials are selected based on their ability to manipulate light. In summary, the physical properties of materials—such as density, thermal conductivity, melting and boiling points, elasticity, and optical properties—are all rooted in the behavior and interactions of particles as described by particle theory. Understanding these properties is essential for designing and selecting materials for various applications across different fields, from engineering and chemistry to optics and construction. By leveraging the insights provided by particle theory, scientists and engineers can develop materials with tailored properties to meet specific needs, driving innovation and advancement in numerous industries.
Technological Innovations and Future Directions
Technological innovations are pivotal in advancing our understanding and applications of particle theory, driving significant future directions. The development of sophisticated particle accelerators, such as the Large Hadron Collider (LHC), has enabled scientists to probe the fundamental nature of matter and energy at unprecedented scales. These accelerators accelerate particles to nearly the speed of light, allowing for collisions that reveal insights into the Higgs boson, dark matter, and other elusive particles. Advanced computational tools and machine learning algorithms are also crucial, as they facilitate the analysis of vast datasets generated by these experiments, helping researchers to identify patterns and anomalies that might otherwise go unnoticed. In the realm of detection technology, advancements in sensor materials and electronics have improved the sensitivity and precision of particle detectors. For instance, the use of silicon-based detectors in high-energy physics experiments has significantly enhanced the ability to track and measure particle interactions. Furthermore, innovations in quantum computing hold promise for simulating complex particle interactions more accurately than classical computers, potentially leading to breakthroughs in understanding quantum field theories and beyond the Standard Model physics. Looking ahead, future directions in technological innovation will likely involve the integration of artificial intelligence (AI) and machine learning (ML) more deeply into experimental design and data analysis. AI can optimize experimental parameters in real-time, maximizing the efficiency of data collection and reducing the time required for discoveries. Additionally, advancements in materials science could lead to the development of new detector technologies with even higher resolution and sensitivity. The implications of these technological advancements are far-reaching. They not only deepen our understanding of the universe at its most fundamental level but also have practical applications across various fields. For example, medical imaging technologies like PET scans and cancer treatments using proton therapy owe their existence to principles derived from particle physics. Moreover, the technological spin-offs from particle physics research have contributed significantly to fields such as materials science, electronics, and computing. In conclusion, technological innovations are the linchpin for advancing particle theory and its applications. As we continue to push the boundaries of what is possible with new technologies, we open up new avenues for scientific discovery and practical innovation. The future of particle theory is thus intricately linked with ongoing technological advancements, promising a rich landscape of exploration and application that will continue to shape our understanding of the universe and improve human lives.