What Is Shear Force
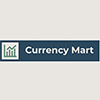
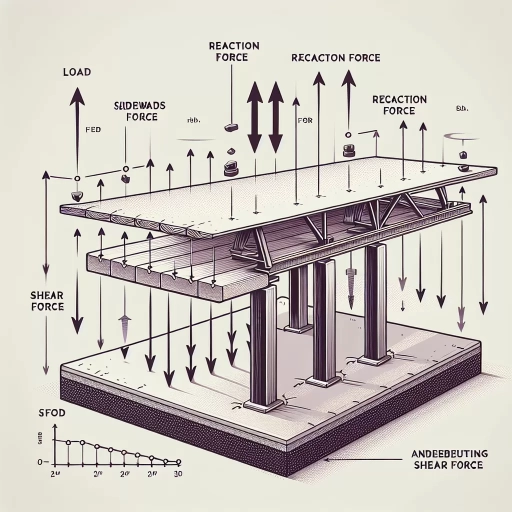
Shear force, a fundamental concept in physics and engineering, plays a crucial role in understanding the behavior of materials under stress. It is the force that causes a material to deform by sliding along a plane parallel to the direction of the force. This phenomenon is ubiquitous in various aspects of our lives, from the structural integrity of buildings to the mechanics of everyday objects. In this article, we will delve into the intricacies of shear force, starting with **Understanding Shear Force Fundamentals**, where we explore the basic principles and definitions that govern this force. We will then examine **Applications and Examples of Shear Force**, highlighting how it manifests in real-world scenarios and its significance in different fields. Finally, we will discuss **Calculating and Analyzing Shear Force**, providing insights into the methods used to quantify and analyze this force in practical contexts. By grasping these concepts, readers will gain a comprehensive understanding of shear force and its importance in both theoretical and practical applications. Let us begin by **Understanding Shear Force Fundamentals**.
Understanding Shear Force Fundamentals
Understanding shear force fundamentals is crucial in various fields, including engineering, physics, and materials science. Shear force, a type of force that causes deformation by sliding along a plane parallel to the direction of the force, plays a pivotal role in the design and analysis of structures, materials, and mechanical systems. To grasp the complexities of shear force, it is essential to delve into its **definition and basic concepts**, which form the foundation of understanding how shear forces operate and their impact on different materials. Additionally, recognizing the **types of shear forces**—such as static and dynamic shear—helps in identifying the specific conditions under which these forces act. Furthermore, understanding the **key factors influencing shear force**, including material properties, geometry, and loading conditions, is vital for predicting and mitigating the effects of shear stress in real-world applications. By exploring these aspects, this article aims to provide a comprehensive overview of shear force fundamentals, equipping readers with the knowledge necessary to apply these principles effectively in their respective fields. Transitioning into the heart of the matter, let us begin by understanding shear force fundamentals.
Definition and Basic Concepts
Understanding shear force fundamentals begins with a clear grasp of its definition and basic concepts. Shear force, a fundamental principle in mechanics and engineering, is defined as the force that causes a material to deform by sliding along a plane parallel to the direction of the force. This type of force is distinct from tensile or compressive forces, which act perpendicular to the material's surface. In practical terms, shear force is what you experience when you try to cut through a piece of paper with scissors or when you twist a metal rod. At its core, shear force involves the application of a force that results in shear stress, which is measured as force per unit area. Shear stress (\(\tau\)) is calculated using the formula \(\tau = \frac{F}{A}\), where \(F\) is the applied force and \(A\) is the area over which the force is applied. The resulting deformation due to shear stress can lead to changes in shape without altering the volume of the material. This is crucial in various engineering applications, such as designing beams, bridges, and mechanical components that must withstand both static and dynamic loads. Key concepts associated with shear force include shear strain, which quantifies the deformation caused by shear stress. Shear strain (\(\gamma\)) is defined as the ratio of the displacement to the original length of the material and is often expressed in radians. Another critical concept is the shear modulus (G), also known as the modulus of rigidity, which describes a material's resistance to shear deformation. The relationship between shear stress and shear strain is given by Hooke's Law for shear, \(\tau = G\gamma\), providing a linear relationship between stress and strain within the elastic limit of the material. Understanding these basic concepts is essential for analyzing and predicting the behavior of structures under various loading conditions. For instance, in civil engineering, knowing how to calculate and manage shear forces is vital for ensuring the structural integrity of buildings and bridges. Similarly, in mechanical engineering, understanding shear forces helps in designing gears, shafts, and other components that are subject to torsional loads. In summary, grasping the definition and basic concepts of shear force is foundational to understanding its role in engineering and mechanics. By recognizing how shear forces act on materials and structures, engineers can design safer, more efficient systems that withstand real-world stresses and strains. This knowledge not only enhances the performance of engineered systems but also ensures their reliability and longevity under diverse operational conditions.
Types of Shear Forces
Shear forces are a fundamental concept in mechanics and engineering, playing a crucial role in understanding the behavior of materials under various types of loading. There are several key types of shear forces that engineers and scientists must consider when designing structures, analyzing material properties, and predicting failure modes. **1. Static Shear Force:** This type of shear force is constant over time and does not change in magnitude or direction. It is commonly encountered in beams and columns where the load is evenly distributed. For instance, a beam supported at both ends with a uniform load applied along its length experiences static shear forces that are highest at the supports and zero at the midpoint. **2. Dynamic Shear Force:** Unlike static shear forces, dynamic shear forces vary with time due to changing loads or vibrations. These forces are critical in the design of structures subjected to seismic activity, wind loads, or other dynamic conditions. For example, during an earthquake, buildings experience dynamic shear forces that can lead to structural failure if not properly accounted for. **3. Torsional Shear Force:** This type of shear force arises from twisting or torsion applied to a member. It is particularly relevant in the design of shafts and axles where rotational loads are prevalent. Torsional shear forces cause shear stress to develop in a circular pattern around the axis of the member, which can lead to failure if the material's shear strength is exceeded. **4. Impact Shear Force:** Impact shear forces occur when a sudden load is applied to a structure, such as during a collision or drop. These forces are transient but can be extremely high, leading to immediate failure if the material does not have sufficient toughness and ductility. Understanding impact shear forces is essential in designing safety features like crash structures in vehicles. **5. Frictional Shear Force:** Frictional shear forces arise from the interaction between two surfaces in contact. This type of force is crucial in understanding phenomena such as sliding friction and rolling resistance. In engineering applications, frictional shear forces are often managed through lubrication or surface treatments to reduce wear and tear on moving parts. Each type of shear force presents unique challenges and considerations for engineers. By understanding these different types, professionals can design more robust and reliable structures that withstand various loading conditions. Whether it's ensuring the stability of buildings under seismic activity or optimizing the performance of mechanical components under dynamic loads, recognizing and addressing the specific characteristics of each type of shear force is paramount for achieving safe and efficient designs. This comprehensive understanding not only enhances structural integrity but also contributes to the overall reliability and longevity of engineered systems.
Key Factors Influencing Shear Force
Understanding the key factors that influence shear force is crucial for grasping the fundamental principles of mechanics and structural integrity. Shear force, a component of the internal forces that act on a material or structure, arises from external loads that cause deformation by sliding along a plane parallel to the direction of the force. Several critical factors contribute to the magnitude and distribution of shear forces. 1. **External Loads**: The primary factor influencing shear force is the type and magnitude of external loads applied to the structure. These loads can be static (e.g., weight) or dynamic (e.g., vibrations, impacts). The greater the load, the higher the shear force experienced by the material. 2. **Material Properties**: The inherent properties of the material, such as its shear modulus, tensile strength, and yield strength, significantly affect how it responds to shear forces. Materials with higher shear moduli are more resistant to deformation under shear stress. 3. **Cross-Sectional Area**: The cross-sectional area of a beam or column directly impacts its ability to resist shear forces. A larger cross-sectional area generally reduces the shear stress because the force is distributed over a greater area. 4. **Beam Length and Support Conditions**: The length of a beam and its support conditions (e.g., simply supported, cantilevered) influence how shear forces are distributed along its span. For instance, simply supported beams typically experience maximum shear forces at their supports. 5. **Load Distribution**: The manner in which loads are distributed across a structure also plays a significant role. Concentrated loads tend to create higher localized shear stresses compared to uniformly distributed loads. 6. **Geometric Shape**: The geometric shape of a structure can affect its susceptibility to shear forces. For example, rectangular beams are generally more resistant to shear than circular ones due to their larger moment of inertia about the neutral axis. 7. **Boundary Conditions**: The constraints imposed by boundary conditions (e.g., fixed ends, pinned ends) can alter the shear force distribution within a structure. Fixed ends, for instance, can increase the shear force near supports due to additional moment constraints. 8. **Dynamic Effects**: In dynamic loading scenarios, factors such as frequency and amplitude of vibrations can significantly influence shear forces by introducing cyclic loading conditions that may lead to fatigue failure over time. Understanding these key factors allows engineers and designers to predict and mitigate potential failures in structures subjected to various types of loading conditions, ensuring safety and efficiency in construction and mechanical design applications. By analyzing these influences comprehensively, one can develop robust designs that withstand both static and dynamic shear forces effectively.
Applications and Examples of Shear Force
Shear force, a fundamental concept in physics and engineering, plays a crucial role in various fields by influencing the structural integrity and performance of materials and systems. This article delves into the diverse applications and examples of shear force, highlighting its significance across different disciplines. In **Structural Engineering and Building Design**, shear force is essential for ensuring the stability and safety of buildings, bridges, and other infrastructure. It helps engineers design structures that can withstand external loads and stresses. In **Mechanical Engineering and Machine Components**, understanding shear force is vital for the design and operation of machinery, as it affects the durability and efficiency of gears, bearings, and other mechanical parts. Additionally, **Natural Phenomena and Environmental Impact** illustrate how shear forces shape our environment, from the movement of tectonic plates to the erosion of landscapes. By exploring these applications, this article aims to provide a comprehensive understanding of shear force, ultimately leading to a deeper appreciation of its underlying fundamentals in **Understanding Shear Force Fundamentals**.
Structural Engineering and Building Design
In the realm of structural engineering and building design, understanding shear force is crucial for ensuring the stability and integrity of structures. Shear force, a lateral force that causes a structure to deform by sliding along a plane parallel to the direction of the force, plays a pivotal role in various aspects of building design. Structural engineers must meticulously analyze and manage shear forces to prevent structural failure, particularly in high-rise buildings, bridges, and other complex structures. For instance, in the design of high-rise buildings, shear forces are significant due to wind loads and seismic activities. Engineers use techniques such as bracing systems and shear walls to resist these forces. Shear walls, often constructed from reinforced concrete or steel, are designed to absorb and distribute lateral loads efficiently, thereby preventing excessive deformation or collapse. Similarly, in bridge construction, shear forces are critical due to the dynamic loads imposed by traffic and environmental conditions. Here, engineers employ innovative designs like box girder bridges or cable-stayed bridges that are optimized to withstand shear stresses. In addition to these large-scale applications, shear force considerations are also essential in everyday building design. For example, in residential construction, shear forces can arise from wind pressure on walls and roofs. To mitigate this, builders use techniques such as nailing patterns and bracing to ensure that the structure can resist these lateral loads without compromising its integrity. Furthermore, in seismic zones, buildings are designed with specific shear-resistant features such as moment-resisting frames and seismic isolation systems to protect against earthquake-induced shear forces. The application of shear force analysis extends beyond traditional building structures to include innovative designs like skyscrapers with unique geometries and sustainable buildings. For instance, the Burj Khalifa in Dubai features a Y-shaped floor plan that helps distribute shear forces more evenly throughout the structure, enhancing its stability against extreme wind conditions. Similarly, sustainable buildings often incorporate green roofs which impose additional shear loads due to soil and vegetation weight; thus, engineers must carefully design these structures to handle these loads while maintaining their ecological benefits. In summary, understanding and managing shear forces is a cornerstone of structural engineering and building design. By leveraging advanced materials, innovative designs, and rigorous analytical techniques, engineers can create structures that are not only aesthetically pleasing but also safe and durable under various loading conditions. This meticulous approach ensures that buildings stand the test of time and environmental challenges, providing secure habitats for occupants while showcasing human ingenuity in engineering.
Mechanical Engineering and Machine Components
Mechanical engineering, a cornerstone of modern technology, heavily relies on the understanding and application of shear force in the design and operation of machine components. Shear force, which causes deformation by sliding along a plane parallel to the direction of the force, is a critical factor in ensuring the structural integrity and performance of various mechanical systems. In mechanical engineering, shear force plays a pivotal role in the analysis and design of machine elements such as gears, shafts, beams, and bolts. For instance, in gear design, shear force is crucial as it affects the teeth's ability to withstand loads without failing. Engineers must carefully calculate the shear stress on gear teeth to ensure they can handle the torque and rotational forces involved. Similarly, in shaft design, shear force is essential for determining the diameter and material properties required to resist torsional loads without excessive deformation or failure. Beams, another common machine component, experience shear forces that can lead to bending and deflection; understanding these forces is vital for designing beams that can support loads safely. Bolts and fasteners also rely on shear force calculations to ensure they can securely hold parts together under various loading conditions. In these applications, engineers often use materials with high shear strength to prevent failure. Additionally, in the context of mechanical systems like engines and transmissions, understanding shear forces helps in optimizing component durability and efficiency. For example, in engine crankshafts, shear forces due to rotational and axial loads must be managed to prevent premature wear or catastrophic failure. Moreover, the application of shear force extends beyond traditional mechanical components to advanced technologies such as robotics and aerospace engineering. In robotics, precise control over shear forces is necessary for grippers and manipulators to handle delicate tasks without causing damage. In aerospace engineering, the high-stress environment of aircraft and spacecraft necessitates meticulous consideration of shear forces in structural components like wings and fuselage. In summary, mechanical engineers must have a deep understanding of shear force to design and develop reliable machine components that can withstand various operational stresses. By applying principles related to shear force, engineers can create more efficient, durable, and safe mechanical systems across a wide range of industries. This fundamental concept underpins many of the technological advancements we see today and continues to drive innovation in mechanical engineering.
Natural Phenomena and Environmental Impact
Natural phenomena, such as earthquakes, landslides, and hurricanes, illustrate the profound impact of shear force on the environment. Shear force, a type of force that causes deformation by sliding along a plane parallel to the direction of the force, plays a crucial role in shaping our planet's surface. During earthquakes, shear forces within the Earth's crust cause tectonic plates to move past each other, resulting in seismic waves that can lead to devastating ground shaking and structural damage. This movement not only reshapes landscapes but also triggers secondary disasters like tsunamis and soil liquefaction, which further exacerbate environmental degradation. Landslides are another example where shear forces are pivotal. When the shear stress on a slope exceeds its strength, it can lead to catastrophic failures such as rockfalls or mudslides. These events can alter ecosystems by blocking rivers, burying habitats, and disrupting nutrient cycles. For instance, a landslide can create a natural dam that alters water flow patterns, affecting aquatic life and potentially leading to downstream flooding when the dam fails. Hurricanes and typhoons demonstrate how shear forces influence atmospheric conditions. The rotation of these storms generates strong winds that exert significant shear forces on coastal areas and inland regions. These forces can cause widespread destruction by uprooting trees, demolishing buildings, and eroding coastlines. The storm surge associated with these events also highlights the power of shear forces in water; as winds push against the surface of the ocean, they create waves that can inundate coastal communities and disrupt marine ecosystems. In addition to these dramatic events, shear forces are constantly at work in more subtle ways. For example, in river systems, shear forces between flowing water and riverbanks contribute to erosion and sediment transport. This process shapes river courses over time and influences the formation of deltas and floodplains—critical habitats for numerous species. Understanding the role of shear force in these natural phenomena is essential for mitigating their environmental impacts. By studying how shear forces act on different materials and systems, scientists can develop strategies for earthquake-resistant construction, landslide prevention measures, and storm-resistant infrastructure. Moreover, recognizing the ongoing effects of shear forces helps in managing natural resources sustainably and predicting future environmental changes. In conclusion, the study of shear force provides valuable insights into the dynamics behind various natural phenomena and their environmental consequences. By applying this knowledge across different fields—from geology to engineering—we can better prepare for and respond to these events, ultimately reducing their adverse impacts on our planet's ecosystems and human communities.
Calculating and Analyzing Shear Force
Calculating and analyzing shear force is a fundamental aspect of engineering and physics, crucial for understanding the structural integrity and behavior of various materials and systems. Shear force, which causes deformation by sliding along a plane parallel to the direction of the force, is a key factor in designing and optimizing structures such as bridges, buildings, and mechanical components. To comprehensively grasp shear force, it is essential to delve into three critical areas: **Mathematical Formulas and Equations**, which provide the theoretical framework for calculating shear forces; **Experimental Methods for Measurement**, which involve practical techniques to measure and validate these forces; and **Software Tools and Simulation Techniques**, which enable detailed analysis and prediction of shear force effects through computational models. By exploring these facets, engineers and researchers can gain a deeper understanding of how shear forces operate and how they impact different systems. This article aims to provide a thorough exploration of these topics, ultimately leading to a robust **Understanding of Shear Force Fundamentals**.
Mathematical Formulas and Equations
When delving into the realm of calculating and analyzing shear force, a deep understanding of mathematical formulas and equations is paramount. Shear force, a critical component in structural engineering and mechanics, is the force that causes a material to deform by sliding along a plane parallel to the direction of the force. The mathematical underpinnings of shear force analysis are rooted in several key formulas and equations. At the heart of shear force calculations lies the beam theory, which involves the use of differential equations to describe the behavior of beams under various loads. One of the fundamental equations in this context is the Euler-Bernoulli beam equation, which relates the deflection of a beam to its loading conditions. This equation is given by \(EI \frac{d^4 y}{dx^4} = q(x)\), where \(E\) is the modulus of elasticity, \(I\) is the moment of inertia, \(y\) is the deflection, \(x\) is the position along the beam, and \(q(x)\) is the distributed load. Another crucial formula is the shear force diagram equation, which can be derived from the equilibrium equations. For a beam subjected to external loads, the shear force \(V(x)\) at any point can be calculated using the relationship \(V(x) = \int q(x) dx\), where \(q(x)\) is the load intensity. This integral represents the cumulative effect of all loads up to a given point. In addition to these, the bending moment equation \(M(x) = \int V(x) dx\) is essential for understanding how shear forces translate into bending moments, which are critical for determining the structural integrity of beams. The interplay between shear forces and bending moments is encapsulated in the relationship \(V = \frac{dM}{dx}\), highlighting how changes in bending moment directly influence shear force. For more complex scenarios involving multiple loads and supports, the method of sections and the principle of superposition are employed. These methods involve breaking down the structure into simpler components and applying equilibrium equations to each part. The resulting system of equations can then be solved to determine the shear forces at various points along the beam. In summary, calculating and analyzing shear force relies heavily on a robust understanding of mathematical formulas and equations. From the Euler-Bernoulli beam equation to shear force diagrams and bending moment calculations, these mathematical tools provide engineers with the necessary framework to predict and mitigate the effects of shear forces in various structural elements. By mastering these equations, engineers can ensure that structures are designed to withstand the stresses imposed by external loads, thereby safeguarding their integrity and functionality.
Experimental Methods for Measurement
When calculating and analyzing shear force, experimental methods play a crucial role in providing accurate and reliable data. These methods involve direct measurement or indirect estimation of shear forces under various conditions, ensuring that theoretical calculations are validated and refined. One of the primary experimental techniques is the use of strain gauges. These tiny sensors are attached to the surface of materials or structures and measure the deformation caused by shear forces. By converting this deformation into electrical signals, strain gauges provide precise quantitative data that can be analyzed to determine the magnitude and distribution of shear forces. Another significant method is the application of load cells and force transducers. These devices are integrated into test setups to directly measure the forces applied to a specimen or structure. For instance, in a torsion test, a load cell can measure the twisting force (torque) applied to a shaft, from which the shear force can be calculated using the geometry of the specimen. Additionally, optical methods such as digital image correlation (DIC) and laser interferometry offer non-contact measurement techniques. DIC involves capturing images of a specimen under load and analyzing pixel displacements to determine strain fields, while laser interferometry measures surface displacements with high precision. Experimental setups often include fixtures and jigs designed to apply controlled loads in specific directions, ensuring that the measured forces are purely shear. For example, in a shear test rig, specimens are clamped between two plates that move relative to each other, simulating pure shear conditions. The data collected from these experiments can then be compared with theoretical models to validate assumptions and improve predictive accuracy. Furthermore, advanced techniques like finite element analysis (FEA) are frequently used in conjunction with experimental methods. FEA allows for the simulation of complex loading scenarios and provides detailed insights into stress distributions within materials. By comparing FEA results with experimental data, researchers can refine their models and enhance their understanding of how shear forces affect different materials and structures. In summary, experimental methods for measuring shear force are essential for validating theoretical calculations and ensuring the accuracy of structural analyses. Through the use of strain gauges, load cells, optical measurement techniques, and carefully designed test setups, engineers can gather comprehensive data that helps in understanding and predicting the behavior of materials under shear loading conditions. This integration of experimental and analytical approaches is crucial for designing safe, efficient, and reliable structures across various engineering disciplines.
Software Tools and Simulation Techniques
When calculating and analyzing shear force, the integration of advanced software tools and simulation techniques is crucial for achieving accurate and reliable results. These tools enable engineers to model complex structures and simulate various loading conditions, allowing for a detailed understanding of how shear forces distribute across different components. Software such as finite element analysis (FEA) tools like ANSYS, ABAQUS, or OpenFOAM, are widely used in this context. These programs allow users to create detailed 3D models of structures, apply different types of loads, and analyze the resulting stresses and strains, including shear forces. Simulation techniques, particularly those based on computational mechanics, provide a robust framework for predicting the behavior of materials under shear loading. For instance, computational fluid dynamics (CFD) can be employed to study the shear forces in fluid dynamics problems, such as in pipeline design or aerodynamics. Similarly, molecular dynamics simulations can offer insights into the material properties at the atomic level, helping in understanding how shear forces affect the microstructure of materials. The use of these software tools and simulation techniques not only enhances the precision of shear force calculations but also facilitates iterative design improvements. By simulating different scenarios and analyzing the outcomes, engineers can optimize structural designs to better withstand shear forces, thereby improving safety and reducing the risk of failure. Additionally, these tools support educational purposes by providing interactive learning environments where students can visualize and experiment with various engineering problems related to shear force. In practical applications, software tools like MATLAB and Python libraries such as NumPy and SciPy are often used for data analysis and visualization of shear force data. These platforms allow for the automation of repetitive tasks, such as data processing and plotting, which is essential for large-scale engineering projects. Furthermore, cloud-based simulation services offer scalability and accessibility, enabling teams to collaborate more effectively on complex projects involving shear force analysis. In summary, the combination of advanced software tools and sophisticated simulation techniques is indispensable for accurately calculating and analyzing shear forces. These technologies not only enhance the accuracy of engineering calculations but also streamline the design process, facilitate educational learning, and support collaborative project management. By leveraging these resources, engineers can ensure that structures are designed to safely withstand various loading conditions, ultimately contributing to improved safety standards in engineering practice.