What Is Ls
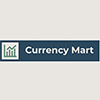
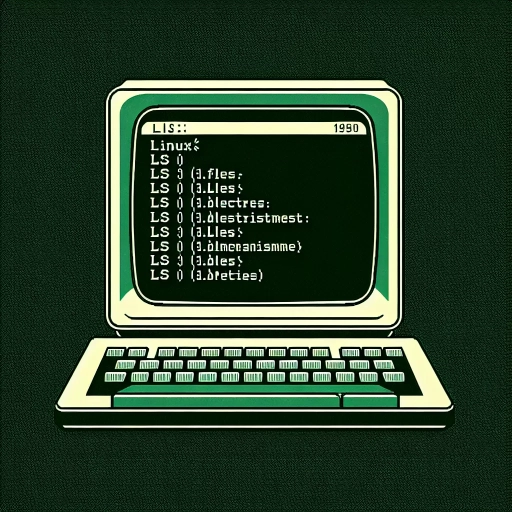
In the realm of advanced technologies, Laser Technology (LS) stands out as a versatile and powerful tool that has revolutionized various industries. From precision manufacturing to medical treatments, LS has become an indispensable asset due to its accuracy, efficiency, and adaptability. This article delves into the multifaceted world of LS, exploring its fundamental principles, diverse applications, and the exciting innovations on the horizon. First, we will **Understanding the Basics of LS**, uncovering the science behind how lasers work and their key characteristics. Next, we will examine **Applications and Uses of LS**, highlighting how this technology is transforming fields such as healthcare, aerospace, and consumer electronics. Finally, we will look ahead to **Future Developments and Innovations in LS**, discussing the potential breakthroughs that could further expand its capabilities. By grasping these core aspects, readers will gain a comprehensive understanding of LS and its profound impact on modern society. Let's begin by **Understanding the Basics of LS**.
Understanding the Basics of LS
Understanding the basics of LS (Laser Scanning) is crucial for anyone interested in advanced surveying and mapping technologies. This article delves into the fundamental aspects of LS, providing a comprehensive overview that is both informative and engaging. To grasp the full scope of LS, it is essential to explore its definition and origins, which lay the groundwork for understanding its core principles. Additionally, examining the key components and features of LS technology will help in appreciating its capabilities and limitations. Finally, a historical context and evolution of LS will reveal how this technology has developed over time, influencing various fields such as engineering, architecture, and environmental science. By starting with the definition and origins of LS, we can establish a solid foundation for further exploration into its components, features, and historical development. This approach ensures a thorough understanding of how LS has become an indispensable tool in modern surveying and mapping practices. Let us begin by defining what LS is and tracing its origins to understand its foundational roots.
Definition and Origins
**Understanding the Basics of LS: Definition and Origins** The term "LS" can refer to several concepts, but in the context of automotive and mechanical engineering, it specifically pertains to the General Motors (GM) LS engine family. The LS engine series is a line of V8 and V6 gasoline engines that have been widely used in various GM vehicles since their introduction in the late 1990s. To grasp the significance and impact of these engines, it's crucial to delve into their definition and origins. **Definition:** The LS engine family is characterized by its all-aluminum construction, which significantly reduces weight compared to traditional iron-block engines. This design choice enhances power-to-weight ratio, contributing to improved performance and fuel efficiency. The LS engines are known for their robust architecture, featuring a deep-skirt block design that provides exceptional strength and durability. They also incorporate advanced technologies such as coil-on-plug ignition, variable valve timing (VVT), and sequential fuel injection, which enhance combustion efficiency and reduce emissions. **Origins:** The development of the LS engine series began in the mid-1990s as part of General Motors' effort to modernize its powertrain offerings. The first generation of LS engines, known as the Gen III, was introduced in 1997 with the launch of the C5 Corvette. This initial iteration set the stage for future generations by introducing key innovations like the all-aluminum block and head design. Over the years, subsequent generations (Gen IV, Gen V, and Gen VI) have built upon these foundational elements, incorporating new technologies such as direct fuel injection, cylinder deactivation, and advanced materials to further enhance performance and efficiency. The success of the LS engine family can be attributed to its versatility; these engines have been used across a wide range of GM vehicles, from high-performance sports cars like the Corvette to more practical applications in trucks and SUVs. Their popularity extends beyond OEM use as well; LS engines have become a favorite among enthusiasts and aftermarket builders due to their ease of modification and robust performance potential. In summary, understanding the definition and origins of the LS engine family provides a solid foundation for appreciating its impact on automotive engineering. From their innovative design features to their widespread adoption across various vehicle platforms, the LS engines represent a significant milestone in GM's history and continue to influence modern engine design. This knowledge is essential for anyone looking to delve deeper into the world of automotive technology and performance enhancement.
Key Components and Features
When delving into the basics of the `ls` command, it is crucial to understand its key components and features. The `ls` command, short for "list," is a fundamental tool in Unix and Unix-like operating systems that allows users to view the contents of directories. At its core, `ls` provides a list of files and directories within a specified directory, but its versatility extends far beyond this basic functionality. ### Key Components 1. **Command Syntax**: The basic syntax of the `ls` command is straightforward: `ls [options] [file|directory]`. Here, options are used to customize the output, and specifying a file or directory is optional; if omitted, it defaults to the current working directory. 2. **Options**: Options are where the power of `ls` truly lies. Common options include `-l` for a detailed (long) listing, `-a` to include hidden files, `-d` to list directories themselves rather than their contents, and `-h` for human-readable file sizes. These options can be combined to tailor the output according to user needs. 3. **File and Directory Information**: When using the `-l` option, `ls` displays detailed information about each file or directory. This includes permissions, number of links, owner and group names, size in bytes, timestamp of last modification, and the name of the file or directory. ### Features 1. **Customizable Output**: One of the standout features of `ls` is its ability to customize output through various options. For example, using `-S` sorts files by size, while `-t` sorts them by modification time. The `-r` option reverses the order of sorting. 2. **Color Coding**: Many modern implementations of `ls`, such as those found in GNU/Linux distributions, support color coding. This feature can be enabled using the `--color=auto` option or by setting an alias in your shell configuration file. Color coding helps differentiate between files and directories at a glance. 3. **Recursive Listing**: The `-R` option allows for recursive listing of directories and their contents. This is particularly useful when you need to see the structure of nested directories without having to navigate through them manually. 4. **Sorting and Filtering**: Beyond basic sorting options like size and time, advanced sorting can be achieved using tools like `sort` in combination with `ls`. For instance, piping the output of `ls` to `sort -n` sorts files numerically by size. 5. **Integration with Other Commands**: The output of `ls` can be piped into other commands for further processing. For example, combining `ls` with `grep` allows you to filter files based on specific patterns or names. In summary, understanding the key components and features of the `ls` command empowers users to efficiently manage and navigate their file systems. By mastering its various options and integrations with other commands, users can tailor their workflow to meet specific needs, making it an indispensable tool in any Unix-like environment. Whether you're a beginner or an advanced user, grasping these fundamentals will significantly enhance your productivity and command-line experience.
Historical Context and Evolution
Understanding the historical context and evolution of LS (Linux Shell) is crucial for grasping its significance and functionality. The journey of LS begins with the early days of Unix, a multi-user, multi-tasking operating system developed in the late 1960s by Bell Labs. The first Unix shell, known as the Thompson shell, was introduced in 1971 by Ken Thompson. This shell provided basic command-line functionality but lacked many features that are now considered essential. The next significant milestone was the introduction of the Bourne shell in 1977 by Stephen Bourne. The Bourne shell, also known as `sh`, added substantial improvements such as job control, input/output redirection, and conditional statements. It became the standard shell for Unix systems and laid the groundwork for future shell developments. In the 1980s, the C shell (`csh`) and Korn shell (`ksh`) were developed. The C shell, created by Bill Joy at the University of California, Berkeley, introduced features like command history and job control that made it more user-friendly. The Korn shell, developed by David Korn at Bell Labs, combined elements from both the Bourne and C shells, offering a more comprehensive set of features. The advent of Linux in the early 1990s marked a pivotal point in the evolution of shells. Linux, an open-source operating system created by Linus Torvalds, adopted the GNU Project's Bash (Bourne-Again SHell) as its default shell. Bash, developed by Brian Fox and later maintained by Chet Ramey, integrated features from various predecessors while adding new functionalities such as programmable completion and improved scripting capabilities. Throughout its evolution, LS has been influenced by user needs and technological advancements. Modern shells like Zsh (Z shell) and Fish (Friendly Interactive Shell) have emerged with enhanced features such as better auto-completion, syntax highlighting, and improved usability. These shells continue to evolve with community contributions, reflecting the dynamic nature of open-source software development. In summary, understanding LS requires a grasp of its historical roots in Unix and its subsequent evolution through various shell iterations. From the basic Thompson shell to the sophisticated modern shells like Bash, Zsh, and Fish, each step has contributed to the robust command-line interface that users rely on today. This historical context not only enriches one's appreciation for LS but also provides a solid foundation for mastering its intricacies and leveraging its full potential.
Applications and Uses of LS
The applications and uses of Life Sciences (LS) are diverse and far-reaching, impacting various sectors in profound ways. At the heart of these applications are three key areas: Industrial and Manufacturing Uses, Medical and Healthcare Applications, and Environmental and Sustainability Roles. In the industrial and manufacturing sphere, LS plays a crucial role in developing innovative technologies and processes that enhance efficiency and productivity. For instance, biotechnology is used to create bio-based products, such as biofuels and bioplastics, which are more sustainable alternatives to traditional materials. In the medical and healthcare domain, LS drives advancements in diagnostics, treatments, and personalized medicine, significantly improving patient outcomes. Additionally, LS contributes to environmental sustainability by providing solutions for pollution control, conservation, and the development of eco-friendly technologies. Understanding these multifaceted applications is essential for appreciating the full impact of LS on modern society. Let's delve into the Industrial and Manufacturing Uses of LS first, exploring how this field is revolutionizing the way we produce goods and services.
Industrial and Manufacturing Uses
Industrial and manufacturing uses of LS (Lithium Sulfur) batteries are poised to revolutionize the energy landscape by offering a more efficient, sustainable, and powerful alternative to traditional battery technologies. One of the primary advantages of LS batteries in industrial settings is their high energy density, which significantly outperforms conventional lithium-ion batteries. This increased capacity allows for longer operational times and reduced battery replacements, making them ideal for applications such as electric forklifts, automated guided vehicles (AGVs), and other heavy-duty machinery. In manufacturing, LS batteries can be integrated into smart factory systems to enhance energy efficiency and reduce downtime. For instance, they can power advanced robotics and IoT devices that require consistent and reliable energy sources. The improved cycle life of LS batteries also means fewer interruptions for recharging or replacement, thereby increasing overall productivity and reducing maintenance costs. Moreover, the environmental benefits of LS batteries are particularly appealing in industrial contexts. Unlike lithium-ion batteries that contain cobalt—a resource often associated with ethical and environmental concerns—LS batteries use sulfur, which is abundant and less harmful to extract. This shift towards more sustainable materials aligns with the growing emphasis on corporate social responsibility and green manufacturing practices. Additionally, the thermal stability of LS batteries makes them safer for use in high-temperature environments common in many industrial processes. This stability reduces the risk of thermal runaway and fires, enhancing workplace safety and compliance with stringent regulatory standards. In terms of scalability, LS battery technology is being developed to meet the diverse needs of various industries. From powering small-scale IoT devices to large-scale industrial equipment, these batteries offer flexibility and adaptability that can be tailored to specific applications. For example, in renewable energy systems, LS batteries can be used for grid-scale energy storage, helping to stabilize power grids and ensure a smooth transition between different energy sources. Overall, the integration of LS batteries into industrial and manufacturing sectors promises significant improvements in efficiency, sustainability, and safety. As research continues to advance this technology, it is likely that LS batteries will become a cornerstone in the transition towards more efficient and environmentally conscious industrial practices. By leveraging these advancements, industries can not only reduce their carbon footprint but also enhance operational efficiency, driving innovation and growth in a rapidly evolving global market.
Medical and Healthcare Applications
Medical and healthcare applications of Laser Technology (LS) have revolutionized the field, offering precision, efficiency, and innovative solutions that enhance patient care and outcomes. One of the most significant uses of LS in medicine is in surgical procedures. Laser surgery allows for precise incisions with minimal bleeding and tissue damage, reducing recovery time and scarring. For instance, laser-assisted cataract surgery has become a preferred method due to its accuracy and reduced risk of complications. Similarly, laser treatments are widely used in dermatology for skin rejuvenation, hair removal, and treating various skin conditions such as acne, scars, and vascular lesions. In the realm of diagnostics, LS plays a crucial role in imaging techniques like Optical Coherence Tomography (OCT), which provides high-resolution images of internal structures without the need for invasive procedures. This is particularly beneficial in ophthalmology for diagnosing retinal diseases and in cardiology for assessing vascular health. Additionally, laser-induced fluorescence spectroscopy helps in early cancer detection by identifying abnormal cellular changes. Laser therapy is also integral in pain management and physical therapy. Low-level laser therapy (LLLT) or photobiomodulation (PBM) stimulates cellular processes to promote tissue repair, reduce inflammation, and alleviate pain. This non-invasive treatment is often used for conditions such as arthritis, tendinitis, and post-surgical recovery. Furthermore, LS is pivotal in medical research and development. Laser-based techniques like laser-induced breakdown spectroscopy (LIBS) enable the analysis of biological samples without sample preparation, aiding in the study of diseases at the molecular level. Moreover, laser microdissection allows researchers to isolate specific cells or tissues from complex biological samples, facilitating detailed genetic and proteomic analyses. The integration of LS with other technologies like robotics has further expanded its applications. Robotic-assisted laser surgery enhances precision and dexterity during complex procedures, improving surgical outcomes. This synergy between technologies underscores the evolving nature of medical and healthcare applications of LS. In summary, the versatility of LS in medical and healthcare settings spans from precise surgical interventions to advanced diagnostic imaging and therapeutic treatments. Its ability to enhance precision, reduce recovery times, and improve patient outcomes makes it an indispensable tool in modern medicine. As research continues to advance, the potential for new and innovative applications of LS in healthcare will only continue to grow.
Environmental and Sustainability Roles
In the realm of environmental and sustainability roles, Life Sciences (LS) plays a pivotal role in addressing some of the most pressing global challenges. As a multidisciplinary field that encompasses biology, ecology, chemistry, and more, LS provides the scientific foundation for understanding and mitigating the impact of human activities on the environment. One of the key applications of LS is in biodiversity conservation. By studying the intricate relationships between species and their ecosystems, scientists can develop strategies to protect endangered species and preserve natural habitats. For instance, genetic analysis can help in identifying and tracking populations of threatened species, while ecological studies inform habitat restoration projects. LS also drives innovation in sustainable agriculture. Through advances in plant genetics and biotechnology, scientists are developing crop varieties that are more resilient to climate change, require fewer resources, and produce higher yields. This not only enhances food security but also reduces the environmental footprint of agricultural practices. Additionally, LS informs sustainable water management by understanding the biological processes that affect water quality and availability. For example, microbiological research helps in developing effective wastewater treatment systems and monitoring water pollution. In the context of climate change, LS contributes significantly to understanding and mitigating its effects. By studying the carbon cycle and microbial processes, scientists can develop new technologies for carbon sequestration and bioenergy production. Furthermore, LS underpins the development of sustainable materials and products. Biodegradable plastics, biofuels, and other bioproducts are being engineered to replace traditional materials that contribute to pollution and waste accumulation. The role of LS in environmental policy and regulation is equally crucial. Scientific data generated from LS research informs policy decisions related to environmental protection, public health, and resource management. For instance, toxicological studies help in setting safety standards for chemicals and pollutants, while epidemiological research links environmental exposures to health outcomes. Moreover, LS fosters community engagement and education on environmental issues. By translating complex scientific findings into accessible information, scientists can empower communities to take action in protecting their local environments. This includes initiatives such as citizen science projects where volunteers contribute to data collection and monitoring efforts. In summary, the applications of Life Sciences in environmental and sustainability roles are vast and multifaceted. From conserving biodiversity to developing sustainable technologies, LS provides the scientific backbone for addressing global environmental challenges. As we continue to face new and evolving threats to our planet's health, the importance of LS in driving sustainable solutions will only continue to grow.
Future Developments and Innovations in LS
As we delve into the future developments and innovations in Life Sciences (LS), it is clear that this field is on the cusp of a transformative era. The advancements in technology and materials are set to revolutionize the way we approach healthcare, research, and diagnostics. Emerging trends and market opportunities are opening up new avenues for growth and collaboration, while also presenting unique challenges that need to be addressed. However, these innovations also raise important ethical considerations that must be carefully navigated to ensure that progress is made responsibly. In this article, we will explore these three critical aspects: the cutting-edge technologies and materials that are driving change, the emerging trends and market opportunities that are shaping the industry, and the challenges and ethical considerations that accompany such rapid progress. Let us begin by examining the **Advancements in Technology and Materials**, which are laying the groundwork for a future where precision medicine, advanced diagnostics, and personalized treatments become the norm.
Advancements in Technology and Materials
Advancements in technology and materials are pivotal in driving the future developments and innovations in Laser Sintering (LS). Over the past few decades, significant strides have been made in both hardware and software, as well as in the development of new materials, which have collectively enhanced the capabilities and applications of LS. On the technological front, advancements in laser systems have led to higher precision and speed. For instance, the introduction of high-power lasers with improved beam quality has enabled faster processing times without compromising on part quality. Additionally, advancements in scanner technology, such as galvanometer scanners and polygon scanners, have increased the scanning speed and accuracy, allowing for more complex geometries to be produced efficiently. In terms of software, sophisticated algorithms and simulation tools now enable better process control and optimization. These tools can predict and mitigate potential issues during the sintering process, ensuring consistent part quality and reducing the need for trial-and-error approaches. Furthermore, the integration of artificial intelligence (AI) and machine learning (ML) into LS systems is revolutionizing the field by enabling real-time monitoring and adaptive process adjustments. This not only improves productivity but also enhances the reliability of the sintering process. Material science has also seen substantial progress, expanding the range of materials available for LS. Traditional polymers such as PA12 and PA11 continue to be popular due to their mechanical properties and ease of processing. However, recent developments have introduced new materials like metal-filled polymers, carbon fiber-reinforced polymers, and even biodegradable polymers. These advanced materials open up new application areas for LS, including aerospace, automotive, medical devices, and sustainable products. The development of functional materials with tailored properties—such as conductive or magnetic properties—further broadens the scope of LS applications. Moreover, the emergence of hybrid materials that combine different types of powders or incorporate nanoparticles is pushing the boundaries of what can be achieved with LS. These hybrid materials offer unique properties that cannot be obtained with single-component powders, such as improved thermal conductivity or enhanced mechanical strength. The ability to customize material properties at a microscale level through LS is particularly beneficial in fields like biomedical engineering where tailored biomaterials can be designed for specific tissue engineering applications. In conclusion, the interplay between technological advancements and material innovations is driving the future of LS. As these technologies continue to evolve, we can expect even more sophisticated applications across various industries. The integration of AI, ML, and advanced simulation tools will further optimize the LS process, while new materials will unlock previously unexplored possibilities. This synergy between technology and materials ensures that LS remains a cutting-edge manufacturing technique capable of meeting the complex demands of modern engineering and beyond.
Emerging Trends and Market Opportunities
As we delve into the future developments and innovations in Life Sciences (LS), it becomes evident that emerging trends and market opportunities are poised to revolutionize the industry. One of the most significant trends is the integration of artificial intelligence (AI) and machine learning (ML) into various facets of LS. AI-driven analytics are enhancing drug discovery by identifying potential candidates more efficiently and predicting their efficacy and safety profiles. Additionally, AI is being leveraged in personalized medicine, enabling tailored treatment plans based on individual genetic profiles and medical histories. This not only improves patient outcomes but also opens up new avenues for targeted therapies. Another burgeoning trend is the rise of gene editing technologies, particularly CRISPR/Cas9. These tools offer unprecedented precision in modifying genetic material, holding immense promise for treating genetic disorders and developing novel therapeutic approaches. The market potential for gene editing is vast, with applications ranging from basic research to clinical treatments, making it a highly attractive area for investment and innovation. The increasing focus on precision medicine is also driving significant advancements. With the help of advanced genomics and proteomics, researchers are able to understand disease mechanisms at a molecular level, leading to more effective and targeted treatments. This shift towards precision medicine is creating new market opportunities in diagnostics, where companies are developing sophisticated tests that can identify biomarkers for specific diseases, allowing for early intervention and better patient management. Furthermore, the convergence of digital health technologies with traditional LS practices is transforming patient care. Wearable devices and mobile health apps are generating vast amounts of data that can be analyzed to monitor health trends, predict disease onset, and optimize treatment regimens. Telemedicine platforms are also expanding access to healthcare services, especially in underserved regions, thereby addressing healthcare disparities. Sustainability and environmental considerations are becoming integral to LS innovations as well. The development of biodegradable materials and green manufacturing processes is gaining traction, reducing the environmental footprint of pharmaceutical production. This trend aligns with growing consumer demand for eco-friendly products and is likely to influence future market dynamics. Lastly, the COVID-19 pandemic has accelerated the adoption of mRNA technology, which has shown remarkable efficacy in vaccine development. This technology has the potential to revolutionize vaccine production by offering rapid response capabilities to emerging infectious diseases. The success of mRNA vaccines has opened up new market opportunities for companies specializing in this area, driving further research and investment into mRNA-based therapies. In summary, the future of LS is characterized by a confluence of technological advancements, shifting market dynamics, and evolving consumer demands. As AI, gene editing, precision medicine, digital health, sustainability, and mRNA technologies continue to evolve, they will create numerous market opportunities and drive innovation within the industry. These trends not only promise to improve healthcare outcomes but also offer significant economic potential, making LS an exciting and dynamic field for both researchers and investors alike.
Challenges and Ethical Considerations
As we delve into the future developments and innovations in Life Sciences (LS), it is crucial to address the challenges and ethical considerations that accompany these advancements. The rapid evolution of LS technologies, such as gene editing tools like CRISPR, precision medicine, and artificial intelligence in healthcare, presents both immense opportunities and significant ethical dilemmas. One of the primary challenges is ensuring that these innovations are accessible and equitable. The high cost of cutting-edge treatments can create a divide between those who can afford them and those who cannot, exacerbating existing health disparities. This raises ethical questions about fairness and justice in healthcare distribution. Another critical issue is the potential for misuse of advanced technologies. For instance, gene editing technologies could be exploited for non-therapeutic purposes, such as creating "designer babies," which raises profound ethical concerns about human identity and the boundaries of scientific intervention. Additionally, the integration of AI in healthcare must be carefully managed to prevent biases in decision-making algorithms that could lead to discriminatory outcomes. Privacy and data security are also significant concerns. The increasing reliance on big data and genomics in LS research necessitates robust safeguards to protect patient information from unauthorized access or misuse. This is particularly pertinent given the sensitive nature of genetic data, which could have far-reaching implications for individuals and their families. Furthermore, there is a need for transparent and inclusive regulatory frameworks to govern these innovations. Regulatory bodies must strike a balance between fostering innovation and protecting public safety, ensuring that new technologies are rigorously tested and validated before they are introduced into clinical practice. Public engagement and education are also essential to build trust and address societal concerns about the ethical implications of these advancements. In conclusion, while future developments in LS hold great promise for improving human health and quality of life, they must be approached with a keen awareness of the ethical challenges involved. Addressing these considerations proactively will be crucial for ensuring that these innovations benefit society as a whole while respecting individual rights and dignity. By fostering a culture of ethical responsibility and transparency, we can harness the full potential of LS to create a healthier, more equitable future for all.