What Is A Physical System
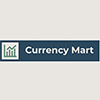
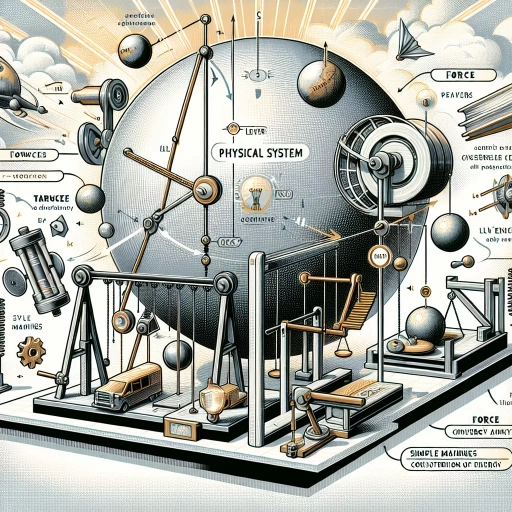
A physical system is a fundamental concept in various scientific disciplines, including physics, engineering, and biology. It refers to a set of interconnected components that interact with each other and their environment, governed by physical laws. Understanding what constitutes a physical system is crucial for analyzing and predicting the behavior of complex phenomena. This article will delve into the definition and scope of a physical system, exploring its key characteristics and properties, and providing examples of real-world applications. By examining these aspects, we will gain a comprehensive insight into how physical systems operate and their significance in everyday life. To begin, let's define the scope and boundaries of what we consider a physical system. --- **Definition and Scope of a Physical System**
Definition and Scope of a Physical System
A physical system, at its core, is a set of components that interact with each other and their environment, governed by physical laws. Understanding the definition and scope of a physical system is crucial for various scientific and engineering disciplines. This article delves into the fundamental aspects of physical systems, starting with the **Basic Components and Boundaries**, which define the structure and limits of these systems. It then explores the **Types of Physical Systems**, highlighting the diverse range of systems from mechanical and electrical to thermal and biological. Finally, it discusses the **Interdisciplinary Applications**, showcasing how physical systems are integral to fields such as physics, engineering, and even biology. By examining these components, types, and applications, we gain a comprehensive view of how physical systems operate and their significance in modern science and technology. This foundational knowledge is essential for designing, analyzing, and optimizing physical systems, making it a cornerstone of scientific inquiry and innovation. Therefore, let us begin by dissecting the basic components and boundaries that form the backbone of any physical system.
Basic Components and Boundaries
In the context of defining and understanding a physical system, it is crucial to identify its basic components and boundaries. A physical system consists of various elements that interact with each other to form a cohesive whole. These components can be categorized into several types: **subsystems**, which are smaller systems within the larger system; **interfaces**, where interactions between subsystems occur; and **environment**, the external surroundings that influence the system's behavior. The **subsystems** are the fundamental building blocks of a physical system. For example, in a car engine, the fuel system, ignition system, and cooling system are all subsystems that work together to enable the engine to function. Each subsystem has its own set of components and processes that contribute to the overall operation of the system. **Interfaces** are critical as they define how different subsystems communicate and exchange energy, matter, or information. In the case of a car engine, the interface between the fuel system and the combustion chamber ensures that fuel is delivered precisely when needed for combustion. These interfaces can be physical connections, such as pipes or wires, or they can be more abstract, like software protocols in a computer system. The **environment** of a physical system includes everything outside the system that affects its operation. This can include external forces like gravity, temperature fluctuations, or other external systems that interact with it. For instance, a refrigerator's performance is influenced by the ambient temperature of its environment. **Boundaries** are essential for defining what is included within the system and what is part of the environment. They help in distinguishing between internal processes and external influences. In a heating system, for example, the boundary might include all components from the boiler to the radiators but exclude external factors like room insulation or window quality. Understanding these basic components and boundaries is vital for analyzing, designing, and optimizing physical systems. By clearly defining what constitutes the system and its interactions with the environment, engineers and scientists can predict behavior, identify potential issues, and make improvements to enhance efficiency and performance. Moreover, recognizing these elements allows for a systematic approach to problem-solving. For instance, diagnosing issues in a complex system like an aircraft requires identifying which subsystems are malfunctioning and how they interact with other parts of the system. This holistic view ensures that solutions address not just symptoms but underlying causes. In summary, the basic components—subsystems, interfaces, and environment—and boundaries of a physical system are fundamental to its definition and scope. These elements provide a structured framework for understanding how systems operate, interact with their surroundings, and can be optimized for better performance. By meticulously defining these aspects, one can gain a comprehensive insight into the workings of any physical system.
Types of Physical Systems
Physical systems, which are defined as collections of physical components that interact with each other and their environment, can be categorized into several distinct types based on their characteristics and functions. **Mechanical Systems**, for instance, involve components such as gears, levers, and springs that convert energy from one form to another. These systems are fundamental in engineering and are used in machines like engines, robots, and mechanical tools. **Thermal Systems** focus on the transfer of heat energy and are crucial in applications like refrigeration, air conditioning, and power generation. **Electrical Systems** consist of components such as circuits, wires, and electronic devices that process and transmit electrical energy; they are essential in modern technology, powering everything from household appliances to complex industrial machinery. **Fluid Systems**, which include hydraulic and pneumatic systems, utilize liquids or gases to transmit energy and are commonly used in automotive systems, industrial processes, and medical devices. **Biological Systems**, while often studied in the context of living organisms, can also be considered physical systems when examining the mechanical, thermal, and electrical processes within living beings. For example, the human circulatory system can be analyzed as a fluid system, while the nervous system can be viewed as an electrical system. **Control Systems**, which integrate various types of physical systems, are designed to regulate and manage the behavior of other systems through feedback mechanisms; they are vital in automation, robotics, and aerospace engineering. Each type of physical system has its own set of principles and methodologies for design, analysis, and optimization, making them indispensable in a wide range of scientific and engineering disciplines. Understanding these different types is crucial for developing innovative solutions to real-world problems and for advancing technological capabilities.
Interdisciplinary Applications
Interdisciplinary applications are a cornerstone in the study and understanding of physical systems, as they leverage insights from multiple fields to provide a comprehensive and nuanced perspective. By integrating principles from physics, mathematics, engineering, biology, and computer science, researchers can tackle complex problems that transcend traditional disciplinary boundaries. For instance, in the realm of biomechanics, engineers and biologists collaborate to analyze the mechanical properties of living tissues, leading to advancements in medical devices and surgical techniques. Similarly, in environmental science, physicists and ecologists work together to model climate dynamics and predict the impacts of human activities on ecosystems. The interdisciplinary approach also enhances the development of smart materials and technologies, where chemists, physicists, and materials scientists combine their expertise to create innovative materials with tailored properties. Furthermore, computational models derived from interdisciplinary research enable simulations that predict the behavior of physical systems under various conditions, aiding in the design of more efficient systems in fields such as energy production and transportation. This holistic approach not only enriches our understanding of physical systems but also fosters innovative solutions that address real-world challenges more effectively than any single discipline could alone. By embracing interdisciplinary applications, we can unlock new avenues for research, drive technological advancements, and ultimately improve our ability to analyze and interact with the physical world around us.
Key Characteristics and Properties
Understanding the key characteristics and properties of physical systems is crucial for grasping the fundamental principles that govern their behavior. This article delves into three critical aspects: Energy and Momentum Conservation, Thermodynamic Principles, and Dynamical Behavior and Equilibrium. Each of these components plays a vital role in comprehending how systems interact and evolve over time. Energy and Momentum Conservation highlight the laws that dictate the balance and transfer of energy and momentum within closed systems, ensuring that these quantities remain constant. Thermodynamic Principles explain how energy transformations occur, including the concepts of entropy and the laws of thermodynamics. Dynamical Behavior and Equilibrium focus on the motion and stability of systems, detailing how they reach and maintain equilibrium states. By exploring these interconnected ideas, we can gain a deeper insight into the underlying mechanics of physical phenomena. This article will begin by examining the foundational concept of Energy and Momentum Conservation, setting the stage for a comprehensive understanding of the other key characteristics and properties.
Energy and Momentum Conservation
In the context of physical systems, the conservation of energy and momentum is a fundamental principle that underpins the behavior and interactions within these systems. **Energy Conservation** states that the total energy of an isolated system remains constant over time, though it can transform between different forms such as kinetic, potential, thermal, and electromagnetic. This principle is encapsulated by the first law of thermodynamics, which asserts that energy cannot be created or destroyed, only converted from one type to another. For instance, in a mechanical system, the sum of kinetic and potential energies remains constant unless external forces act upon it. **Momentum Conservation**, on the other hand, is governed by Newton's third law of motion and is a direct consequence of the homogeneity of space. It states that the total momentum of a closed system (a system not affected by external forces) is constant over time. Momentum is the product of an object's mass and velocity, and in collisions or interactions within a closed system, the total momentum before the interaction must equal the total momentum after the interaction. This principle is crucial in understanding phenomena such as elastic and inelastic collisions, where the conservation of momentum helps predict the final velocities of objects involved. Together, these principles provide a robust framework for analyzing and predicting the behavior of physical systems. They are key characteristics because they are universally applicable and invariant under different reference frames, making them cornerstone principles in physics. For example, in celestial mechanics, the conservation of energy and momentum allows astronomers to predict the orbits of planets and stars with high accuracy. Similarly, in particle physics, these principles help explain the outcomes of high-energy collisions and the properties of subatomic particles. The conservation laws also highlight the interplay between different forms of energy and momentum within a system. For instance, in a car engine, chemical energy stored in fuel is converted into kinetic energy of the vehicle, illustrating how energy transforms while maintaining its total value. In a similar vein, during a rocket launch, the momentum of expelled gases equals the momentum gained by the rocket itself, demonstrating momentum conservation in action. Understanding these principles is essential for designing and optimizing physical systems across various fields, from engineering to astrophysics. They serve as foundational tools for problem-solving and prediction, ensuring that any analysis or model adheres to the fundamental laws governing the behavior of physical systems. Thus, the conservation of energy and momentum stands as a cornerstone characteristic that defines and governs the dynamics of all physical systems.
Thermodynamic Principles
Thermodynamic principles are fundamental to understanding the behavior of physical systems, particularly in terms of energy and its interactions. At the core of thermodynamics are four laws that govern how energy is transformed and conserved within a system. The **Zeroth Law of Thermodynamics** establishes the concept of temperature, allowing for the definition of a temperature scale and enabling the comparison of thermal states between different systems. The **First Law**, also known as the law of energy conservation, states that energy cannot be created or destroyed, only converted from one form to another. This principle is crucial for understanding the balance between internal energy, work, and heat transfer within a system. The **Second Law of Thermodynamics** introduces the concept of entropy, a measure of disorder or randomness. It asserts that in any spontaneous process, the total entropy of an isolated system will always increase, reflecting the directionality of natural processes. This law also introduces the idea of reversible and irreversible processes, with reversible processes being idealized scenarios where entropy remains constant. The **Third Law of Thermodynamics** provides a basis for absolute zero, the theoretical temperature at which all matter would have zero entropy. This law is essential for understanding the limits of refrigeration and the behavior of materials at very low temperatures. Key characteristics and properties of thermodynamic systems include **internal energy**, which encompasses all forms of energy within the system; **enthalpy**, which accounts for internal energy plus the energy associated with the pressure and volume of a system; and **entropy**, which quantifies disorder or randomness. **Specific heat capacity** and **latent heat** are important properties that describe how a system responds to temperature changes and phase transitions, respectively. Additionally, **thermodynamic equilibrium** is a state where the system's properties are uniform throughout and do not change over time, indicating that there are no net flows of matter or energy. Understanding these principles and properties is vital for analyzing and predicting the behavior of physical systems under various conditions. For instance, in engineering applications, thermodynamic principles guide the design of engines, refrigerators, and other devices that involve energy conversion. In chemistry, these principles help explain chemical reactions and the stability of compounds. In biology, thermodynamics underpins metabolic processes and the energy transformations that sustain life. By grasping these fundamental laws and characteristics, one can better comprehend how energy interacts within and between physical systems, enabling more accurate predictions and efficient designs across diverse fields.
Dynamical Behavior and Equilibrium
In the context of physical systems, dynamical behavior and equilibrium are fundamental concepts that describe how these systems evolve over time and stabilize under certain conditions. Dynamical behavior refers to the time-dependent changes in a system's state, governed by its internal dynamics and external influences. This can include oscillations, growth, decay, or more complex patterns such as chaos. For instance, in mechanical systems, the motion of a pendulum or the vibration of a spring are examples of dynamical behavior where the system's state changes continuously. Equilibrium, on the other hand, represents a stable state where the net force acting on the system is zero, and there is no net change in its state over time. In thermodynamic systems, equilibrium is achieved when the temperature, pressure, and chemical potential are uniform throughout the system. For example, a gas in a sealed container will eventually reach thermal equilibrium where its temperature is uniform throughout. Key characteristics of dynamical behavior include sensitivity to initial conditions, which can lead to divergent outcomes even from very similar starting points, as seen in chaotic systems. Another characteristic is the presence of attractors—stable states towards which the system tends to evolve over time. Equilibrium states often act as attractors, drawing the system towards stability. Properties of equilibrium states are crucial for understanding the long-term behavior of physical systems. One key property is stability; an equilibrium state is considered stable if small perturbations do not cause the system to deviate significantly from this state. Another property is uniqueness; in some systems, there may be multiple equilibrium states, each corresponding to different stable configurations. The interplay between dynamical behavior and equilibrium is essential for predicting and analyzing the performance of physical systems. For example, in control theory, understanding how a system behaves dynamically allows engineers to design feedback mechanisms that drive the system towards a desired equilibrium state. Similarly, in climate science, studying the dynamical behavior of atmospheric and oceanic systems helps in predicting long-term climate equilibria. In summary, dynamical behavior describes the evolving nature of physical systems over time, while equilibrium represents stable states that these systems may achieve. Understanding these concepts is vital for analyzing and predicting the behavior of various physical systems across different disciplines. By recognizing key characteristics such as sensitivity to initial conditions and attractors in dynamical behavior, and properties like stability and uniqueness in equilibrium states, scientists and engineers can better design, control, and predict the outcomes of complex physical systems.
Examples and Real-World Applications
In the realm of complex systems, understanding and applying principles from various disciplines is crucial for innovation and problem-solving. This article delves into the real-world applications of systems thinking across three pivotal domains: Mechanical Systems in Engineering, Biological Systems in Medicine, and Environmental Systems in Ecology. Each of these areas showcases how a systematic approach can lead to groundbreaking advancements. For instance, mechanical systems in engineering enable the design and optimization of machinery and infrastructure, while biological systems in medicine help in understanding disease mechanisms and developing treatments. Similarly, environmental systems in ecology inform conservation strategies and sustainable practices. By examining these examples, we can appreciate the universal applicability of systems thinking. This article will explore these concepts in depth, starting with the intricate world of Mechanical Systems in Engineering, where precision and efficiency are paramount. Here, we will see how engineers leverage system dynamics to create sophisticated technologies that underpin modern society.
Mechanical Systems in Engineering
Mechanical systems in engineering are intricate assemblies of components designed to perform specific tasks, leveraging principles of mechanics and thermodynamics. These systems are ubiquitous in various industries, including automotive, aerospace, manufacturing, and healthcare. For instance, in the automotive sector, mechanical systems such as engines, transmissions, and braking systems work in harmony to ensure efficient and safe vehicle operation. In aerospace engineering, mechanical systems like propulsion units and control surfaces are critical for aircraft stability and navigation. In manufacturing, mechanical systems including robotic arms and conveyor belts enhance production efficiency and precision. Even in healthcare, mechanical ventilators and prosthetic limbs rely on sophisticated mechanical designs to support patient care. One of the key aspects of mechanical systems is their ability to convert energy from one form to another. For example, an internal combustion engine converts chemical energy from fuel into mechanical energy, which is then transmitted through the drivetrain to propel a vehicle. Similarly, wind turbines convert kinetic energy from wind into electrical energy through a series of mechanical components. These conversions are often achieved through complex interactions between gears, levers, pistons, and other mechanical elements. Real-world applications of mechanical systems also highlight their adaptability and innovation. In renewable energy, mechanical systems are integral to the operation of solar trackers that adjust the angle of solar panels to maximize energy capture. In consumer electronics, mechanical systems such as hard disk drives and cooling fans ensure data storage and device reliability. Additionally, advancements in 3D printing technology rely heavily on precise mechanical movements to create complex structures layer by layer. The design and development of mechanical systems involve rigorous engineering principles, including kinematics, dynamics, and materials science. Engineers use computational models and simulations to optimize system performance, reduce wear and tear, and enhance safety. For example, finite element analysis (FEA) is used to predict stress distributions in mechanical components under various loads, ensuring that they can withstand operational conditions without failure. Moreover, the integration of sensors and actuators with mechanical systems has led to the development of mechatronic systems, which combine mechanical engineering with electronics and software engineering. This integration enables real-time monitoring and control of mechanical processes, improving efficiency and reliability. For instance, in modern vehicles, electronic control units (ECUs) monitor engine performance and adjust parameters in real-time to optimize fuel efficiency and reduce emissions. In summary, mechanical systems are fundamental to engineering across diverse fields, offering a wide range of applications that transform raw energy into useful work. Their design involves a deep understanding of mechanical principles and innovative technologies, making them indispensable for modern industrial and technological advancements.
Biological Systems in Medicine
Biological systems play a crucial role in medicine, serving as the foundation for understanding human health and disease. These systems, which include the circulatory, respiratory, nervous, and immune systems among others, are intricate networks of organs and tissues that work together to maintain homeostasis and overall well-being. In medicine, understanding these biological systems is essential for diagnosing and treating various conditions. For instance, the circulatory system's role in transporting oxygen and nutrients to cells is critical for maintaining tissue health; any disruption, such as atherosclerosis or heart failure, can lead to severe health issues. The immune system's ability to recognize and combat pathogens is vital for preventing infections and autoimmune diseases. Real-world applications include organ transplantation, where understanding the compatibility of biological systems between donor and recipient is crucial for successful outcomes. Additionally, advancements in biotechnology have led to the development of targeted therapies that exploit specific biological pathways to treat diseases like cancer and diabetes. For example, monoclonal antibodies target specific proteins involved in disease processes, while gene therapy aims to correct genetic defects by modifying or replacing genes within cells. These interventions highlight the importance of biological systems in medical research and practice, enabling healthcare professionals to develop more effective treatments and improve patient outcomes. Furthermore, the study of biological systems informs public health strategies, such as vaccination programs that leverage the immune system's capacity to generate long-lasting immunity against infectious diseases. Overall, the integration of knowledge about biological systems into medical practice has revolutionized healthcare, offering new avenues for prevention, diagnosis, and treatment of a wide range of medical conditions.
Environmental Systems in Ecology
Environmental systems in ecology are complex networks that interact to sustain life and regulate the planet's natural processes. These systems include biotic components like plants, animals, and microorganisms, as well as abiotic factors such as water, soil, air, and sunlight. The interactions within these systems are crucial for maintaining ecological balance and supporting biodiversity. For instance, in a forest ecosystem, trees absorb carbon dioxide and release oxygen through photosynthesis, while decomposers break down organic matter to recycle nutrients back into the soil. This interplay ensures that the ecosystem remains healthy and resilient. In real-world applications, understanding environmental systems is vital for managing natural resources sustainably. For example, in agriculture, recognizing the role of pollinators like bees and butterflies helps farmers implement practices that protect these species, thereby maintaining crop yields. Similarly, in urban planning, incorporating green spaces and wetlands can mitigate the effects of urban runoff and improve air quality. Conservation efforts also rely on understanding environmental systems; for instance, restoring wetlands can help protect against flooding by absorbing excess water and filtering pollutants. Moreover, environmental systems play a critical role in climate regulation. The carbon cycle, which involves the exchange of carbon between the atmosphere, oceans, and land, is a key example. Human activities such as deforestation and burning fossil fuels disrupt this cycle by releasing excessive carbon dioxide into the atmosphere, contributing to global warming. Understanding these dynamics allows policymakers to develop strategies for reducing greenhouse gas emissions and promoting carbon sequestration through reforestation and renewable energy initiatives. Additionally, environmental systems are essential for human health. Clean water systems, for example, depend on intact ecosystems that filter and purify water naturally. The loss of these systems due to pollution or habitat destruction can lead to waterborne diseases and other health issues. Similarly, the spread of zoonotic diseases—those transmitted from animals to humans—can be influenced by environmental factors such as deforestation and climate change, which alter animal habitats and increase human-animal interactions. In conclusion, environmental systems are intricate webs of life that underpin ecological health and human well-being. Recognizing their importance and understanding how they function is crucial for addressing pressing environmental challenges such as climate change, biodiversity loss, and sustainable resource management. By integrating this knowledge into policy and practice, we can better protect these vital systems for future generations.