What Is The Heaviest Metal
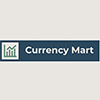
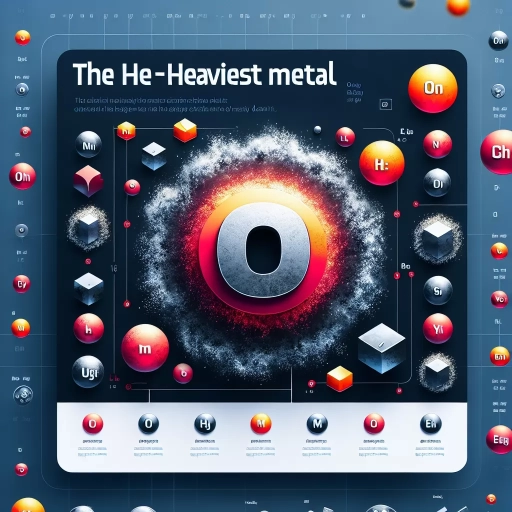
Understanding the Concept of Density in Metals
Understanding the concept of density in metals is a fundamental aspect of materials science, with far-reaching implications across various industrial and scientific applications. Density, defined as the mass per unit volume of a substance, is a critical property that influences the behavior and performance of metals in different contexts. This article delves into three key areas: the definition and measurement of density, the factors that influence metal density, and the importance of density in metal applications. First, we will explore the definition and measurement of density, which involves understanding the precise methods used to determine this property. Accurate measurement is crucial for ensuring the reliability of data and for making informed decisions in engineering and manufacturing processes. Next, we will examine the factors that influence metal density, including chemical composition, crystal structure, and processing conditions. These factors can significantly impact the physical and mechanical properties of metals, making them more or less suitable for specific uses. Finally, we will discuss the importance of density in metal applications, highlighting how this property affects everything from structural integrity to thermal conductivity. By grasping these concepts, engineers and researchers can optimize metal selection and design for a wide range of applications. To begin, let's delve into the definition and measurement of density, a foundational understanding that underpins all subsequent discussions on this critical property.
Definition and Measurement of Density
**Definition and Measurement of Density** Density, a fundamental physical property, is defined as the mass per unit volume of a substance. It is a measure of how tightly packed the molecules of a material are, providing insight into its composition and structure. Mathematically, density (\(\rho\)) is expressed as \(\rho = \frac{m}{V}\), where \(m\) is the mass of the substance and \(V\) is its volume. This relationship highlights that density is independent of the size of the sample, making it a characteristic property of each material. To measure density, one can use several methods. The most straightforward approach involves determining the mass of a sample using a balance and then measuring its volume. For regular shapes, volume can be calculated using geometric formulas. However, for irregular shapes or substances like powders, displacement methods are often employed. In these cases, the volume of water displaced by the sample when submerged in a container can be measured to find the volume of the sample. Another method involves using a pycnometer, a device specifically designed for measuring the volume of solids and liquids with high precision. By filling the pycnometer with water and then adding the sample, the difference in water levels before and after adding the sample gives the volume of the sample. In metals, understanding density is crucial due to its implications on various physical and mechanical properties. For instance, metals with higher densities tend to have higher melting points and greater strength. This is why metals like tungsten and osmium, known for their exceptionally high densities, are used in applications requiring extreme durability and resistance to wear. The measurement of density also plays a significant role in identifying and characterizing different metals. Since each metal has a unique density value, this property can serve as a diagnostic tool in metallurgy. For example, distinguishing between different alloys or detecting impurities within a metal sample can be facilitated by comparing their densities against known values. In summary, density is a critical property that reflects the intrinsic nature of materials, including metals. Its precise measurement through various methods allows for a deeper understanding of material properties and applications, making it an essential concept in fields such as metallurgy and materials science. As we delve into the heaviest metals, comprehending density becomes pivotal in appreciating their unique characteristics and uses.
Factors Influencing Metal Density
When delving into the concept of density in metals, it is crucial to understand the various factors that influence this physical property. Density, defined as mass per unit volume, is a fundamental characteristic that distinguishes metals from one another. Several key factors contribute to the density of metals, each playing a significant role in determining their overall weight and structural integrity. First and foremost, the atomic mass of the metal's constituent elements significantly impacts its density. Metals with higher atomic masses generally have higher densities due to the greater number of protons and neutrons in their atomic nuclei. For instance, osmium, with an atomic mass of approximately 190 u (unified atomic mass units), is one of the densest naturally occurring elements known, boasting a density of about 22.59 g/cm³. In contrast, lighter metals like aluminum (atomic mass around 27 u) have lower densities, with aluminum's density being approximately 2.70 g/cm³. Another critical factor influencing metal density is the crystal structure or lattice arrangement of the atoms. The packing efficiency of the crystal lattice can vary significantly among different metals, affecting how tightly the atoms are packed within the material. For example, metals with face-centered cubic (FCC) or hexagonal close-packed (HCP) structures tend to have higher packing efficiencies compared to those with body-centered cubic (BCC) structures. This variation in packing efficiency directly impacts the overall density of the metal. The presence of impurities or alloying elements can also alter the density of a metal. When other elements are introduced into a metal's crystal lattice, they can either occupy interstitial sites or substitute for host atoms, leading to changes in both the atomic mass and the lattice spacing. These changes can result in either an increase or decrease in density depending on the nature and concentration of the impurities. For instance, adding carbon to iron to form steel can slightly increase its density due to carbon's ability to occupy interstitial sites within the iron lattice. Additionally, processing conditions such as temperature and pressure during manufacturing can influence metal density. Thermal treatments like annealing or quenching can alter the microstructure of metals, affecting their porosity and grain size, which in turn impact their overall density. Similarly, high-pressure processes can compact metals more tightly, reducing any voids or defects that might lower their density. Lastly, the presence of defects within the metal's crystal structure, such as vacancies or dislocations, can also affect its density. These defects can introduce voids or distortions within the lattice, leading to a reduction in the material's overall density. Advanced manufacturing techniques and quality control measures are often employed to minimize such defects and ensure consistent material properties. In summary, understanding the factors that influence metal density—atomic mass, crystal structure, impurities, processing conditions, and defects—is essential for appreciating why certain metals are heavier than others. These factors collectively determine the unique characteristics of each metal, making them suitable for various applications ranging from aerospace engineering to consumer electronics. By recognizing these influences, researchers and engineers can design and develop materials with tailored properties to meet specific needs across diverse industries.
Importance of Density in Metal Applications
The importance of density in metal applications cannot be overstated, as it plays a crucial role in determining the suitability and performance of metals across various industries. Density, defined as mass per unit volume, is a fundamental property that influences the physical and mechanical characteristics of metals. In engineering, for instance, density is critical for structural integrity and weight considerations. For example, in aerospace engineering, lightweight yet strong metals like aluminum and titanium are preferred due to their high strength-to-weight ratio, which is directly related to their density. Conversely, in construction and civil engineering, denser metals such as steel and iron are often chosen for their ability to provide robust support and stability. In the automotive industry, the density of metals affects fuel efficiency and safety. Lower-density materials like magnesium and aluminum alloys are increasingly used to reduce vehicle weight, thereby improving fuel economy and reducing emissions. However, these materials must also meet stringent safety standards, necessitating careful consideration of their density in relation to crashworthiness and structural resilience. Density also impacts the thermal and electrical properties of metals. For instance, copper's high density contributes to its excellent thermal conductivity, making it an ideal material for heat sinks and electrical wiring. Similarly, the high density of tungsten makes it a preferred choice for high-temperature applications such as filaments in incandescent bulbs and rocket nozzles. Furthermore, the economic aspect of metal density is significant. The cost-effectiveness of a metal is often tied to its density; denser metals generally require less material to achieve the same structural strength, which can reduce overall costs. This is particularly important in large-scale industrial applications where material savings can translate into substantial economic benefits. Additionally, the environmental impact of metal density should not be overlooked. The extraction, processing, and transportation of metals are energy-intensive processes that contribute to greenhouse gas emissions. By selecting metals with optimal densities for specific applications, industries can minimize material usage and associated environmental footprints. In scientific research and development, understanding metal density is essential for advancing technologies. For example, in the field of superconductivity, researchers seek materials with specific density profiles to enhance their superconducting properties. Similarly, in nanotechnology, the manipulation of metal densities at the nanoscale allows for the creation of novel materials with unique properties. In conclusion, the importance of density in metal applications is multifaceted and far-reaching. It influences structural integrity, thermal and electrical properties, economic viability, environmental impact, and technological innovation. As industries continue to evolve and demand more efficient and sustainable solutions, a deep understanding of metal density will remain a cornerstone of material science and engineering. By leveraging this knowledge, we can develop better materials that meet the complex needs of modern applications while minimizing adverse effects on the environment.
Identifying the Heaviest Metals Known
Identifying the heaviest metals known is a fascinating journey through the periodic table, where elements with extraordinary properties and significant uses await discovery. This exploration delves into three key areas: an overview of heavy metals in the periodic table, their distinctive properties and characteristics, and examples of the heaviest metals along with their practical applications. Understanding the placement and distribution of heavy metals within the periodic table provides a foundational knowledge of their atomic structures and how these elements are categorized. The properties and characteristics of heavy metals, such as their high atomic masses, dense nature, and often unique chemical behaviors, set them apart from lighter elements. Finally, examining specific examples of the heaviest metals, like Oganesson and Tennessine, reveals their potential uses in scientific research and technological advancements. By grasping these aspects, we can better appreciate the complexity and utility of these heavy metals. Let us begin by examining the overview of heavy metals in the periodic table, where their classification and positioning offer insights into their fundamental nature.
Overview of Heavy Metals in the Periodic Table
Heavy metals, a term often used to describe a group of elements with high atomic numbers and densities, are scattered throughout the periodic table. These elements are typically characterized by their high atomic mass, which often correlates with their position in the periodic table. Starting from the transition metals in the d-block, such as iron (Fe), copper (Cu), and zinc (Zn), heavy metals extend into the actinides and lanthanides, where elements like uranium (U) and thorium (Th) reside. The heaviest metals known are predominantly found in the actinide series, which includes elements from actinium (Ac) to lawrencium (Lr). These elements are highly radioactive and have complex electronic configurations that contribute to their unique properties. One of the defining features of heavy metals is their ability to form ions with multiple charges, which allows them to participate in a wide range of chemical reactions. For instance, mercury (Hg), a heavy metal in group 12, can form ions such as Hg²⁺ and Hg²⁺₂, making it versatile in both biological and industrial contexts. Similarly, lead (Pb), another well-known heavy metal in group 14, exhibits multiple oxidation states, making it useful in applications like batteries and pigments. The physical properties of heavy metals also set them apart. Many are dense and have high melting points, which are advantageous in various industrial applications. Tungsten (W), for example, has the highest melting point among all elements at 3422°C, making it ideal for high-temperature applications such as filaments in incandescent light bulbs. Additionally, heavy metals like gold (Au) and platinum (Pt) are renowned for their high ductility and resistance to corrosion, qualities that make them valuable in jewelry and catalytic converters. Despite their utility, heavy metals pose significant environmental and health risks. Their toxicity can lead to severe health issues when ingested or inhaled, as seen with mercury poisoning and lead exposure. Environmental contamination by heavy metals is a major concern due to their persistence in ecosystems and potential bioaccumulation in organisms. Regulatory bodies around the world have implemented strict guidelines to limit the release of heavy metals into the environment and to ensure safe handling practices. In summary, heavy metals are a diverse group of elements with unique chemical and physical properties that make them both valuable and hazardous. Understanding their characteristics is crucial for harnessing their benefits while mitigating their risks. As we delve into identifying the heaviest metals known, it becomes clear that these elements represent the extremes of the periodic table in terms of atomic mass and complexity, offering insights into the fundamental nature of matter itself.
Properties and Characteristics of Heavy Metals
Heavy metals, a diverse group of elements characterized by their high atomic mass and density, exhibit a range of unique properties and characteristics that distinguish them from lighter elements. These metals, often defined as those with a density greater than 5 g/cm³, include well-known examples such as lead, mercury, and cadmium, as well as less common ones like osmium and iridium. One of the most significant properties of heavy metals is their high atomic mass, which contributes to their dense nature. For instance, osmium, the densest naturally occurring element, has an atomic mass of approximately 190 u (unified atomic mass units) and a density of 22.59 g/cm³, making it nearly twice as dense as lead. Another characteristic of heavy metals is their tendency to form ions with a high charge. This property makes them highly reactive and capable of forming stable compounds with other elements. For example, transition metals like iron and copper can form ions with multiple charges (Fe²⁺ and Fe³⁺ for iron; Cu⁺ and Cu²⁺ for copper), which is crucial for their roles in biological systems and industrial applications. Heavy metals also often exhibit strong metallic bonds, leading to high melting and boiling points. Tungsten, for instance, has the highest melting point among all elements at 3422°C, making it invaluable in high-temperature applications such as filaments in incandescent light bulbs. The electronic configuration of heavy metals is another key aspect. Many heavy metals are transition metals or actinides, which means they have partially filled d or f subshells. This electronic structure allows them to participate in various chemical reactions and form complexes with ligands, which is essential for their catalytic properties. For example, platinum is used extensively in catalytic converters in vehicles due to its ability to facilitate redox reactions without being consumed by the reaction itself. Additionally, heavy metals can be toxic and pose significant environmental and health risks. Lead, mercury, and cadmium are notorious for their harmful effects on human health and ecosystems. Lead exposure can lead to neurological damage and developmental issues, while mercury is known to accumulate in the food chain and cause severe neurological and kidney problems. Cadmium exposure has been linked to kidney damage and certain types of cancer. Despite these risks, heavy metals play critical roles in various industries and biological systems. In nature, some heavy metals are essential micronutrients for plants and animals. For example, zinc is crucial for enzyme function and protein synthesis in humans, while copper is necessary for the production of hemocyanin in certain mollusks. In technology, heavy metals are used in batteries (lead-acid batteries), electronics (copper wiring), and even in medical treatments (gold nanoparticles in cancer therapy). In summary, the properties and characteristics of heavy metals—such as their high density, reactivity, strong metallic bonds, unique electronic configurations, potential toxicity, and essential roles in various applications—make them both fascinating and critical components of our world. Understanding these properties is essential for identifying the heaviest metals known and appreciating their significance across different fields.
Examples of Heaviest Metals and Their Uses
Among the heaviest metals known, several stand out for their unique properties and diverse applications. One of the heaviest metals is **Osmium**, with an atomic mass of approximately 190.23 u (unified atomic mass units). Osmium is the densest naturally occurring element and is used in fountain pen nibs, electrical contacts, and other wear-resistant applications due to its extreme hardness and resistance to corrosion. Another heavy metal is **Iridium**, with an atomic mass of about 192.217 u. Iridium is highly corrosion-resistant and is often used in high-performance alloys for jet engine components, satellite components, and even in the production of crucibles for growing single crystals. **Tungsten**, though not as heavy as osmium or iridium with an atomic mass of around 183.84 u, is still one of the densest elements and has a very high melting point. This makes it ideal for use in filaments for incandescent light bulbs, rocket nozzles, and superalloys for aerospace applications. **Gold**, while not as dense as these metals with an atomic mass of about 196.9665 u, is another heavy metal that finds extensive use due to its malleability, ductility, and resistance to corrosion. Gold is widely used in jewelry, electronics (for contacts and connectors), dental fillings, and even as a catalyst in chemical reactions. **Uranium**, with an atomic mass of approximately 238.0289 u, is one of the heaviest naturally occurring elements and is primarily known for its role in nuclear reactors and atomic bombs due to its radioactive properties. However, it also has applications in the production of radiation shielding and as a catalyst in certain chemical processes. **Platinum**, with an atomic mass of about 195.084 u, is another heavy metal that is highly valued for its catalytic properties and resistance to corrosion. It is used extensively in catalytic converters for vehicles to reduce emissions, in jewelry due to its aesthetic appeal, and in laboratory equipment. These heavy metals play critical roles across various industries due to their unique physical and chemical properties. Their applications range from enhancing the performance of high-tech devices to providing durable materials for everyday use. Understanding these metals' characteristics and uses not only highlights their importance but also underscores the ongoing research into their potential future applications. As technology continues to evolve, the demand for these heavy metals is likely to increase, driving further innovation in extraction methods, alloy development, and sustainable use practices.
Applications and Implications of Heavy Metals
Heavy metals, a group of elements known for their high density and often toxic properties, play a multifaceted role in modern society. Their applications are diverse and widespread, ranging from industrial processes to everyday consumer products. This article delves into the various facets of heavy metals, exploring their industrial uses, environmental and health implications, and the future research and development in this field. On one hand, heavy metals are indispensable in industrial settings due to their unique properties such as conductivity, strength, and resistance to corrosion. For instance, metals like copper and zinc are crucial in electrical wiring and galvanizing steel respectively. However, these industrial applications come with significant environmental and health costs. The release of heavy metals into the environment can lead to pollution of water bodies, soil contamination, and adverse health effects on humans and wildlife. Understanding these implications is essential for developing sustainable practices and mitigating harm. Looking ahead, future research and development in heavy metals will focus on finding safer alternatives, improving recycling technologies, and enhancing environmental remediation methods. This ongoing research aims to balance the economic benefits of heavy metal use with the need for environmental stewardship and public health protection. Transitioning to their industrial uses, it is clear that heavy metals are integral to many manufacturing processes. The next section will explore the specific roles these metals play in various industries and how their unique properties make them irreplaceable in these contexts.
Industrial Uses of Heavy Metals
Heavy metals, known for their high density and often toxic properties, play crucial roles in various industrial applications due to their unique physical and chemical characteristics. One of the most significant uses of heavy metals is in the production of alloys and steels. For instance, tungsten, with its exceptionally high melting point, is used in the manufacture of high-speed tools and wear-resistant parts. Chromium, another heavy metal, is a key component in stainless steel production, enhancing corrosion resistance and durability. Molybdenum, with its ability to withstand extreme temperatures, is integral to the production of high-strength steel alloys used in aerospace and automotive industries. In the realm of electronics, heavy metals such as lead and tin are essential for soldering and plating due to their low melting points and good electrical conductivity. Lead-acid batteries, widely used in automotive and renewable energy systems, rely on lead as a primary component. Cadmium, despite its toxicity, is utilized in rechargeable nickel-cadmium batteries due to its high energy density and long cycle life. The mining and construction sectors also heavily rely on heavy metals. Zinc, for example, is used in galvanizing steel to protect it from corrosion, extending the lifespan of infrastructure such as bridges and buildings. Copper, while not as dense as some other heavy metals, is a critical conductor in electrical wiring and circuits due to its excellent electrical conductivity. In addition to these applications, heavy metals are vital in the production of catalysts. Platinum and palladium, for instance, are used in catalytic converters in vehicles to reduce harmful emissions by converting pollutants into less harmful gases. These metals' ability to facilitate chemical reactions without being consumed makes them indispensable in environmental protection technologies. Moreover, heavy metals have significant implications in healthcare and medical research. Gold, due to its biocompatibility and resistance to corrosion, is used in dental fillings and medical implants. Silver's antimicrobial properties make it useful in wound dressings and medical devices. However, the use of heavy metals also raises concerns about environmental pollution and health risks, necessitating stringent regulations and safe handling practices. Overall, the industrial uses of heavy metals are diverse and critical, contributing significantly to technological advancements and economic development. However, their application must be balanced with careful management to mitigate potential environmental and health impacts. Understanding the applications and implications of heavy metals is essential for harnessing their benefits while ensuring sustainable and responsible use.
Environmental and Health Implications
The environmental and health implications of heavy metals are profound and far-reaching, underscoring the critical need for stringent regulation and responsible management. Heavy metals, such as lead, mercury, arsenic, and cadmium, are known for their persistence in the environment and their ability to bioaccumulate in living organisms. When released into the environment through industrial activities, mining operations, or improper waste disposal, these metals can contaminate soil, water, and air, leading to widespread ecological damage. For instance, mercury emissions from coal-fired power plants can accumulate in aquatic ecosystems, transforming into methylmercury—a potent neurotoxin that disrupts the food chain and poses significant risks to wildlife and human health. Human exposure to heavy metals can occur through various pathways, including ingestion of contaminated food and water, inhalation of polluted air, and dermal contact with tainted soil or products. The health consequences are severe and diverse. Lead exposure, for example, is linked to cognitive impairments, developmental delays, and cardiovascular diseases. Mercury exposure has been associated with neurological disorders, including tremors, memory loss, and birth defects. Arsenic exposure is a known carcinogen, increasing the risk of skin, bladder, and lung cancers. Cadmium exposure can lead to kidney damage and bone demineralization. The cumulative effect of heavy metal pollution on public health is alarming. In communities near industrial sites or areas with poor waste management practices, residents often face elevated levels of heavy metal exposure, exacerbating health disparities and socioeconomic inequalities. Moreover, the economic burden of heavy metal pollution is substantial, encompassing costs related to healthcare, environmental remediation, and lost productivity. From an environmental perspective, heavy metals disrupt ecosystems by altering nutrient cycles and affecting microbial communities. This can lead to a decline in biodiversity as sensitive species are pushed to extinction by the toxic conditions. Additionally, heavy metals can interfere with plant growth and agricultural productivity, impacting food security and sustainable land use practices. Addressing these challenges requires a multifaceted approach that includes stringent regulatory frameworks, advanced technologies for pollution control, and public education campaigns. Governments must enforce strict emissions standards for industries and ensure proper disposal of hazardous waste. Technological innovations such as phytoremediation (using plants to clean up contaminated soil) and advanced filtration systems can help mitigate the impact of heavy metal pollution. Public awareness programs are crucial in educating communities about the risks associated with heavy metals and promoting behaviors that reduce exposure. In conclusion, the environmental and health implications of heavy metals are complex and interwoven, necessitating a comprehensive strategy to manage their use and mitigate their adverse effects. By combining policy interventions, technological solutions, and community engagement, we can work towards a safer and more sustainable future where the risks posed by these metals are significantly reduced.
Future Research and Development in Heavy Metals
Future research and development in heavy metals are poised to revolutionize various sectors, from environmental remediation to advanced materials science. One of the key areas of focus will be the development of more efficient and sustainable methods for extracting heavy metals from ores, minimizing environmental impact while maximizing yield. This could involve the use of biotechnology, where microorganisms are engineered to leach metals from ores more effectively, or the implementation of green chemistry principles to reduce the use of toxic reagents. Another significant area of research will be in the realm of recycling and recovery of heavy metals from waste materials. As the global demand for metals continues to rise, finding ways to recover these valuable resources from electronic waste, batteries, and other discarded products will become increasingly important. Advanced technologies such as hydrometallurgy and electrometallurgy are being explored to improve the efficiency and cost-effectiveness of metal recovery processes. In addition, there is a growing interest in the application of heavy metals in emerging technologies like renewable energy systems and advanced electronics. For instance, heavy metals such as indium and gallium are crucial for the production of solar panels and high-efficiency LEDs. Research into new synthesis methods and material properties will be essential for optimizing these applications. Moreover, the biomedical sector is also expected to see significant advancements through the study of heavy metals. Certain heavy metals like gold, silver, and platinum have shown promise in medical treatments due to their antimicrobial properties and ability to target cancer cells selectively. Future research may uncover new therapeutic uses for these metals or develop safer alternatives that mitigate their potential toxicity. Environmental science will also benefit from ongoing research into the behavior and mitigation of heavy metal pollution. Understanding how heavy metals interact with ecosystems at the molecular level can inform strategies for remediation and prevention of contamination. This includes developing more sensitive analytical techniques for detecting trace amounts of heavy metals in water and soil, as well as exploring phytoremediation—using plants to absorb and remove heavy metals from contaminated sites. Lastly, advances in nanotechnology are opening up new avenues for the application of heavy metals at the nanoscale. Nanoparticles made from heavy metals can exhibit unique properties that are not seen in their bulk form, such as enhanced catalytic activity or improved optical properties. These nanoparticles have potential applications in fields ranging from catalysis to biosensing. In summary, future research and development in heavy metals will be multifaceted, addressing both the challenges associated with their extraction and use while exploring new frontiers in technology and medicine. By leveraging cutting-edge scientific techniques and interdisciplinary approaches, scientists aim to unlock the full potential of these elements while ensuring their safe and sustainable use for generations to come.