What Is Hyperpolarization
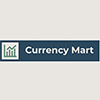
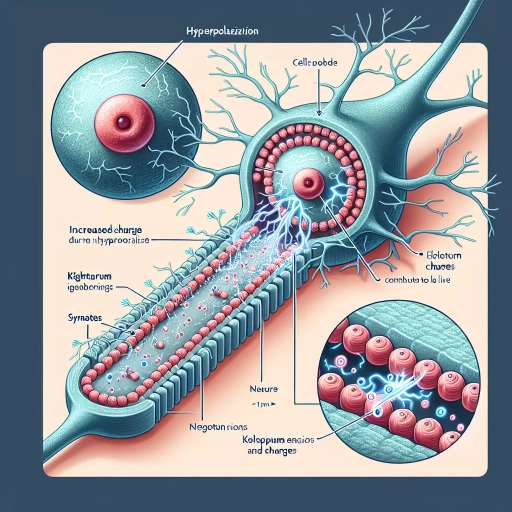
Hyperpolarization is a fascinating phenomenon that has garnered significant attention across various scientific disciplines, including physics, chemistry, and biology. At its core, hyperpolarization refers to the process of enhancing the magnetic polarization of nuclei or electrons beyond the thermal equilibrium limit, leading to enhanced signal-to-noise ratios in magnetic resonance imaging (MRI) and other spectroscopic techniques. This article delves into the intricacies of hyperpolarization, starting with an in-depth exploration of the concept itself, where we will dissect the underlying mechanisms and principles that govern this process. We will then examine the diverse types and examples of hyperpolarization, highlighting their unique characteristics and applications. Finally, we will discuss the far-reaching implications and practical applications of hyperpolarization, from medical diagnostics to materials science. By understanding the concept of hyperpolarization, we lay the foundation for appreciating its multifaceted nature and its potential to revolutionize various fields of research and technology. Therefore, let us begin by understanding the concept of hyperpolarization.
Understanding the Concept of Hyperpolarization
Understanding the concept of hyperpolarization is crucial for grasping various biological and physiological processes. Hyperpolarization, a state where the electrical potential of a cell becomes more negative than its resting potential, plays a significant role in cellular function and communication. This article delves into three key aspects: the definition and mechanism of hyperpolarization, its biological significance, and its historical context. By defining and explaining the mechanisms behind hyperpolarization, we can understand how it affects cellular behavior and signaling pathways. The biological significance of hyperpolarization will be explored in terms of its impact on neural function, muscle contraction, and other physiological processes. Additionally, a historical context will provide insight into how our understanding of hyperpolarization has evolved over time. To begin, let's first explore the definition and mechanism of hyperpolarization, which will lay the groundwork for understanding its broader implications.
Definition and Mechanism
Hyperpolarization is a critical physiological process that occurs in neurons, where the electrical potential across the cell membrane becomes more negative than the resting membrane potential. This phenomenon is essential for regulating neuronal excitability and preventing excessive firing. The mechanism of hyperpolarization involves several key components: 1. **Ion Channels and Pumps**: Hyperpolarization is primarily driven by the movement of ions across the cell membrane. Potassium (K+) channels play a crucial role, as they allow K+ ions to flow out of the cell, increasing the negativity inside. Sodium-potassium pumps also contribute by actively transporting K+ ions into the cell and sodium (Na+) ions out, further enhancing the negative potential. 2. **Inhibitory Synaptic Transmission**: Inhibitory neurotransmitters, such as GABA (gamma-aminobutyric acid) and glycine, bind to receptors on the postsynaptic neuron, opening chloride (Cl-) channels. The influx of Cl- ions into the cell increases the negativity of the membrane potential, leading to hyperpolarization. 3. **Electrical Properties of the Membrane**: The cell membrane's electrical properties, including its capacitance and resistance, influence how quickly and effectively hyperpolarization occurs. The membrane acts as a capacitor, storing electrical charge, while its resistance affects the flow of ions. 4. **Temporal and Spatial Summation**: Hyperpolarization can result from both temporal summation (repeated stimuli over time) and spatial summation (multiple stimuli from different locations). This integration of signals helps in fine-tuning neuronal responses to varying levels of input. 5. **Regulation of Neuronal Firing**: By making the membrane potential more negative, hyperpolarization increases the threshold for action potential generation. This regulatory mechanism prevents neurons from firing excessively, thereby maintaining balanced neural activity and preventing conditions such as seizures or hyperexcitability disorders. Understanding these mechanisms is crucial for grasping how hyperpolarization contributes to the overall functioning of the nervous system, ensuring precise and controlled neuronal communication.
Biological Significance
Hyperpolarization, a phenomenon where the membrane potential of a cell becomes more negative than its resting potential, holds significant biological importance across various physiological processes. At the cellular level, hyperpolarization often serves as a protective mechanism to prevent excessive neuronal firing, thereby maintaining neural homeostasis. For instance, in neurons, hyperpolarization can follow an action potential to ensure a refractory period, during which the neuron is less likely to fire again. This prevents overstimulation and maintains the integrity of neural signaling pathways. In the context of sensory perception, hyperpolarization plays a crucial role in regulating the sensitivity of sensory receptors. For example, in photoreceptors of the retina, light-induced hyperpolarization reduces the release of neurotransmitters, which in turn decreases the signal sent to the brain, allowing for precise light intensity detection. Similarly, in mechanoreceptors responsible for touch and pressure, hyperpolarization helps modulate the sensitivity to mechanical stimuli, ensuring that the nervous system can accurately interpret environmental changes. Furthermore, hyperpolarization is integral to the functioning of certain types of muscle cells. In smooth muscle cells, hyperpolarization can lead to relaxation, which is essential for regulating blood pressure and gastrointestinal motility. This mechanism helps in maintaining vascular tone and ensuring proper digestion by preventing excessive contraction. Additionally, hyperpolarization is involved in synaptic plasticity, a process fundamental to learning and memory. During long-term potentiation (LTP), a form of synaptic strengthening, hyperpolarization can modulate the efficacy of synaptic transmission by altering the excitability of postsynaptic neurons. This dynamic regulation allows for the fine-tuning of neural circuits, which is critical for cognitive functions. In pathophysiological contexts, dysregulation of hyperpolarization mechanisms can lead to various disorders. For example, abnormalities in potassium channels that contribute to hyperpolarization have been linked to conditions such as epilepsy and certain types of arrhythmias. Understanding these mechanisms is crucial for developing targeted therapeutic interventions. In summary, hyperpolarization is not merely a passive electrical phenomenon but a vital regulatory process that underpins numerous biological functions, from maintaining neural homeostasis and sensory perception to modulating muscle activity and synaptic plasticity. Its dysregulation can have profound implications for health, highlighting the importance of continued research into its biological significance.
Historical Context
Understanding the concept of hyperpolarization requires a deep dive into its historical context, which is intricately linked with the development of neuroscience and our understanding of neuronal function. The term "hyperpolarization" itself refers to the process by which the electrical potential of a neuron becomes more negative than its resting membrane potential, typically due to an influx of chloride ions or an efflux of potassium ions. Historically, this concept emerged from early 20th-century research on the electrical properties of neurons. In the early 1900s, scientists like Luigi Galvani and Emil du Bois-Reymond laid the groundwork for understanding electrical signals in biological tissues. However, it was not until the mid-20th century that the detailed mechanisms behind neuronal signaling began to unravel. The work of Alan Hodgkin and Andrew Huxley in the 1950s was pivotal; their experiments on squid giant axons led to the development of the Hodgkin-Huxley model, which described how ions move across neuronal membranes to generate action potentials. The discovery of hyperpolarization as a distinct phenomenon can be traced back to these studies. Hodgkin and Huxley's work showed that neurons have specific ion channels that open and close in response to changes in membrane potential, leading to either depolarization (the process that initiates an action potential) or hyperpolarization (which can inhibit or delay the generation of an action potential). This understanding revolutionized neuroscience by providing a mechanistic basis for how neurons communicate. Further advancements in the field came with the development of patch-clamp techniques in the 1970s by Erwin Neher and Bert Sakmann. This method allowed researchers to study individual ion channels in real-time, providing detailed insights into how hyperpolarization occurs at the molecular level. These studies revealed that hyperpolarization can result from various mechanisms, including the activation of inhibitory neurotransmitter receptors or the opening of specific potassium channels. The historical context also highlights the role of key figures such as John Eccles, who in the 1950s and 1960s conducted extensive research on synaptic transmission and inhibitory postsynaptic potentials (IPSPs), which are often associated with hyperpolarization. Eccles' work demonstrated that hyperpolarization is not just a passive process but an active mechanism that plays a crucial role in regulating neuronal excitability and synaptic plasticity. In summary, understanding hyperpolarization is deeply rooted in a century of scientific inquiry into neuronal function. From the foundational work on electrical properties of tissues to the detailed molecular mechanisms uncovered through advanced techniques, each step has contributed to our current comprehension of how hyperpolarization shapes neuronal communication and behavior. This historical context underscores the importance of continued research into the intricate processes governing neuronal activity, ensuring that our understanding of hyperpolarization remains dynamic and evolving.
Types and Examples of Hyperpolarization
Hyperpolarization, a critical physiological process, involves the increase in the electrical potential difference across a cell's membrane, making it more negative than the resting potential. This phenomenon is crucial in various cell types and plays a significant role in maintaining cellular homeostasis and regulating cellular activities. In neurons, hyperpolarization can inhibit the generation of action potentials, thereby modulating neural communication. In muscle cells, it helps in regulating muscle contraction and relaxation. Additionally, hyperpolarization occurs in other cell types, such as epithelial cells and certain immune cells, where it influences various cellular functions. Understanding these different types of hyperpolarization is essential for grasping the intricate mechanisms that govern cellular behavior. This article will delve into the specifics of neuronal hyperpolarization, muscle cell hyperpolarization, and hyperpolarization in other cell types, starting with the intricate mechanisms of neuronal hyperpolarization.
Neuronal Hyperpolarization
Neuronal hyperpolarization is a critical process in the functioning of neurons, where the electrical potential across the cell membrane becomes more negative than the resting potential. This phenomenon is essential for regulating neuronal excitability and preventing excessive firing, thereby maintaining balanced neural activity. Hyperpolarization can occur through various mechanisms, including the influx of chloride ions or the efflux of potassium ions, often mediated by inhibitory neurotransmitters such as GABA (gamma-aminobutyric acid) and glycine. When an inhibitory neurotransmitter binds to its receptor on the postsynaptic neuron, it opens chloride channels, allowing chloride ions to flow into the cell. This influx of negatively charged ions shifts the membrane potential further away from the threshold for action potential generation, making it more difficult for the neuron to fire. Similarly, potassium channels can be activated, leading to an increased efflux of potassium ions, which also contributes to hyperpolarization. This process is vital for fine-tuning neural responses and ensuring that neurons do not become overly excited, which could lead to pathological conditions such as seizures or neuronal damage. For instance, in the context of synaptic inhibition, hyperpolarization helps in reducing the likelihood of postsynaptic neurons firing in response to excitatory inputs, thus playing a crucial role in shaping neural circuits and modulating information processing within the brain. Understanding neuronal hyperpolarization is key to grasping how neurons integrate and process information, and it has significant implications for understanding neurological disorders where hyperpolarization mechanisms may be disrupted.
Muscle Cell Hyperpolarization
Muscle cell hyperpolarization is a critical physiological process that involves the increase in the electrical potential difference across the muscle cell membrane, making it more negative than its resting state. This phenomenon is essential for regulating muscle activity and preventing excessive contraction. In muscle cells, hyperpolarization can occur through various mechanisms, such as the activation of potassium channels or the inhibition of sodium channels. When potassium channels open, potassium ions (K+) flow out of the cell, increasing the negativity inside the cell membrane. Conversely, if sodium channels are inhibited, fewer sodium ions (Na+) enter the cell, also contributing to hyperpolarization. One key example of muscle cell hyperpolarization is seen in the context of inhibitory neurotransmission. For instance, when an inhibitory neurotransmitter like gamma-aminobutyric acid (GABA) binds to its receptors on muscle cells, it can activate chloride channels or GABA-gated potassium channels. This activation leads to an influx of chloride ions (Cl-) into the cell or an efflux of potassium ions, resulting in hyperpolarization. This hyperpolarized state makes it more difficult for the muscle cell to reach the threshold potential necessary for an action potential, thereby reducing muscle contraction and promoting relaxation. Another example is the role of hyperpolarization in preventing muscle fatigue. During prolonged muscle activity, the accumulation of extracellular potassium ions can lead to depolarization and muscle fatigue. However, certain mechanisms such as the activation of the sodium-potassium pump help to restore the resting membrane potential by pumping potassium ions back into the cell and sodium ions out. Additionally, some neurons release neurotransmitters that induce hyperpolarization in muscle cells, ensuring that muscles do not become overactive and fatigued. In clinical contexts, understanding muscle cell hyperpolarization is crucial for managing conditions like muscle spasms or cramps. Medications that enhance GABAergic transmission or directly activate potassium channels can be used to induce hyperpolarization and alleviate these symptoms. Furthermore, research into the molecular mechanisms of hyperpolarization has led to the development of therapeutic strategies aimed at modulating ion channel activity to treat various neuromuscular disorders. In summary, muscle cell hyperpolarization is a vital process that helps regulate muscle function by preventing excessive contraction and promoting relaxation. Through mechanisms involving ion channels and neurotransmitters, hyperpolarization ensures proper muscle function and prevents conditions such as muscle fatigue and spasms. Its significance extends to clinical applications where understanding and manipulating hyperpolarization can lead to effective treatments for neuromuscular disorders.
Hyperpolarization in Other Cell Types
Hyperpolarization in other cell types extends beyond the well-documented cases in neurons and muscle cells, showcasing its diverse roles across various cellular environments. In **endothelial cells**, which line blood vessels, hyperpolarization can regulate vascular tone by influencing the contraction and relaxation of smooth muscle cells. This is often mediated through the activation of potassium channels, leading to an increase in the negative resting membrane potential. This mechanism is crucial for maintaining blood pressure and ensuring proper blood flow to different tissues. In **epithelial cells**, hyperpolarization plays a significant role in ion transport and fluid balance. For instance, in the kidneys, hyperpolarization of epithelial cells helps in the regulation of sodium and potassium ion transport, which is essential for maintaining electrolyte balance and fluid homeostasis. Similarly, in the respiratory epithelium, hyperpolarization can affect the secretion of mucus and other substances, impacting respiratory health. **Immune cells**, such as T lymphocytes and macrophages, also exhibit hyperpolarization as part of their signaling pathways. During immune responses, hyperpolarization can modulate the activity of these cells by altering their membrane potential, which in turn affects their ability to proliferate, differentiate, or produce cytokines. This electrical signaling complements traditional biochemical pathways to fine-tune immune responses. In **smooth muscle cells**, hyperpolarization is a key mechanism for relaxation, particularly in blood vessels and the gastrointestinal tract. The activation of certain potassium channels leads to an outward flow of potassium ions, resulting in a more negative membrane potential. This hyperpolarization reduces the likelihood of calcium channel opening, thereby decreasing intracellular calcium levels and causing muscle relaxation. Furthermore, **stem cells** and **cancer cells** have been found to exhibit unique patterns of hyperpolarization that influence their proliferation and differentiation. In stem cells, hyperpolarization can signal the transition from a quiescent state to an active state, promoting cell division and differentiation. In cancer cells, altered hyperpolarization patterns can contribute to their abnormal growth and survival by disrupting normal cellular signaling pathways. In summary, hyperpolarization is not limited to neurons and muscle cells but is a widespread phenomenon that plays critical roles in various cell types. It regulates vascular tone, ion transport, immune responses, muscle relaxation, and even the behavior of stem cells and cancer cells. Understanding these diverse functions of hyperpolarization provides insights into cellular physiology and pathophysiology, highlighting its importance across different biological contexts.
Applications and Implications of Hyperpolarization
Hyperpolarization, a technique that significantly enhances the magnetic resonance signal of certain nuclei, has far-reaching applications and implications across various fields. This article delves into the multifaceted benefits of hyperpolarization, exploring its clinical applications, its role in research and diagnostic tools, and its therapeutic potential. In the realm of clinical applications, hyperpolarization is revolutionizing medical imaging by providing high-resolution images of metabolic processes in real-time, enabling early disease detection and personalized treatment plans. The research and diagnostic tools section highlights how hyperpolarization enhances the sensitivity of magnetic resonance imaging (MRI) and spectroscopy, allowing scientists to study biological processes at the molecular level with unprecedented detail. Furthermore, the therapeutic potential of hyperpolarization is being explored for targeted treatments, where hyperpolarized agents can be used to monitor drug delivery and efficacy. By examining these three key areas, this article aims to provide a comprehensive understanding of how hyperpolarization is transforming healthcare and scientific research. Transitioning to the first supporting section, we will explore the clinical applications of hyperpolarization in greater detail.
Clinical Applications
Clinical applications of hyperpolarization are revolutionizing various medical imaging and diagnostic techniques, particularly in the field of magnetic resonance imaging (MRI). Hyperpolarization significantly enhances the signal-to-noise ratio (SNR) of MRI scans, allowing for higher resolution and faster imaging times. This is particularly beneficial in diagnosing diseases such as cancer, where early detection is crucial. For instance, hyperpolarized carbon-13 MRI can highlight metabolic changes in tumors, providing valuable insights into tumor biology and treatment response. In cardiovascular imaging, hyperpolarized MRI can assess myocardial perfusion and function with greater precision, aiding in the diagnosis of heart conditions like coronary artery disease. Additionally, hyperpolarized xenon-129 MRI offers unparalleled visualization of lung function, making it an invaluable tool for diagnosing and monitoring respiratory diseases such as chronic obstructive pulmonary disease (COPD). The enhanced sensitivity also enables real-time monitoring of metabolic processes, which can guide personalized treatment strategies. Furthermore, the non-invasive nature of hyperpolarized MRI reduces the need for ionizing radiation, making it a safer option for patients undergoing repeated scans. Overall, the clinical applications of hyperpolarization are transforming the landscape of medical imaging by providing more accurate, efficient, and patient-friendly diagnostic tools.
Research and Diagnostic Tools
Hyperpolarization, a technique that enhances the magnetic resonance signal of nuclei, has significant implications in various research and diagnostic fields. One of the primary applications is in Magnetic Resonance Imaging (MRI) and Nuclear Magnetic Resonance (NMR) spectroscopy. Hyperpolarized MRI, for instance, allows for the detection of metabolic processes in real-time, which is particularly valuable in oncology for monitoring tumor metabolism and response to treatment. This enhanced sensitivity enables researchers to observe subtle changes that would be undetectable with conventional MRI techniques. In the realm of diagnostics, hyperpolarized carbon-13 (¹³C) MRI has shown promise in detecting early signs of diseases such as cancer and neurological disorders. For example, hyperpolarized ¹³C-labeled pyruvate can be used to monitor the conversion of pyruvate to lactate, a process indicative of cancerous tissue. This method provides a non-invasive means to assess tumor aggressiveness and monitor therapeutic efficacy. Furthermore, hyperpolarization extends its utility into drug development and pharmacokinetics. By hyperpolarizing specific drug molecules or their metabolites, researchers can track their distribution and metabolism within the body with unprecedented detail. This capability aids in understanding drug efficacy, identifying potential side effects, and optimizing drug delivery systems. In addition to these clinical applications, hyperpolarization tools are also crucial in basic scientific research. For instance, hyperpolarized xenon gas can be used to study lung function and structure at the molecular level, providing insights into respiratory diseases such as chronic obstructive pulmonary disease (COPD). Similarly, hyperpolarized water and other solvents are being explored for their potential in studying protein structures and interactions, which is vital for understanding disease mechanisms at the molecular level. The integration of hyperpolarization with other advanced imaging techniques like positron emission tomography (PET) and optical imaging further enhances its diagnostic capabilities. This multimodal approach allows for a more comprehensive understanding of biological processes, enabling better disease diagnosis and treatment planning. In summary, hyperpolarization significantly enhances the sensitivity and specificity of various diagnostic and research tools. Its applications span from clinical diagnostics to basic scientific research, offering new avenues for understanding disease mechanisms, monitoring therapeutic responses, and optimizing drug development processes. As technology continues to evolve, the potential for hyperpolarization to revolutionize healthcare and scientific research is vast and promising.
Therapeutic Potential
Hyperpolarization, a phenomenon that enhances the magnetic resonance signal of certain nuclei, holds significant therapeutic potential across various medical fields. One of the most promising applications is in magnetic resonance imaging (MRI). Hyperpolarized MRI can provide high-resolution images of metabolic processes in real-time, allowing for early detection and monitoring of diseases such as cancer, cardiovascular conditions, and neurological disorders. For instance, hyperpolarized carbon-13 MRI can track the metabolism of glucose in tumors, helping to identify cancerous tissues more accurately and monitor the efficacy of treatments. This technology also has implications for personalized medicine, enabling tailored treatment plans based on individual metabolic profiles. In addition to imaging, hyperpolarization can be used to develop novel therapeutic agents. Hyperpolarized xenon gas, for example, has been explored for its potential in lung imaging and functional assessment. It can help diagnose respiratory diseases like chronic obstructive pulmonary disease (COPD) and asthma more effectively than traditional methods. Furthermore, hyperpolarized xenon's ability to bind to specific proteins makes it a candidate for molecular imaging, which could lead to new diagnostic tools for neurological conditions such as Alzheimer's disease. The therapeutic potential of hyperpolarization extends into the realm of drug development and delivery. By enhancing the signal of specific nuclei within drugs, researchers can track the distribution and metabolism of these compounds in the body. This real-time monitoring can improve our understanding of drug pharmacokinetics and pharmacodynamics, leading to more efficient drug design and reduced side effects. Moreover, hyperpolarized agents could be engineered to target specific tissues or cells, allowing for more precise and effective drug delivery systems. Another area where hyperpolarization shows promise is in the field of regenerative medicine. By using hyperpolarized nuclei to label stem cells or other therapeutic cells, researchers can track their migration, differentiation, and integration into tissues. This capability could significantly advance our understanding of tissue repair mechanisms and the development of cell-based therapies for conditions such as heart disease and spinal cord injuries. In conclusion, the therapeutic potential of hyperpolarization is vast and multifaceted. From enhancing diagnostic capabilities through advanced imaging techniques to improving drug development and delivery, this technology has the potential to revolutionize various aspects of healthcare. As research continues to explore and refine these applications, we can expect significant advancements in patient care and treatment outcomes across a range of medical conditions.