What Is A Solid Structure
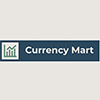
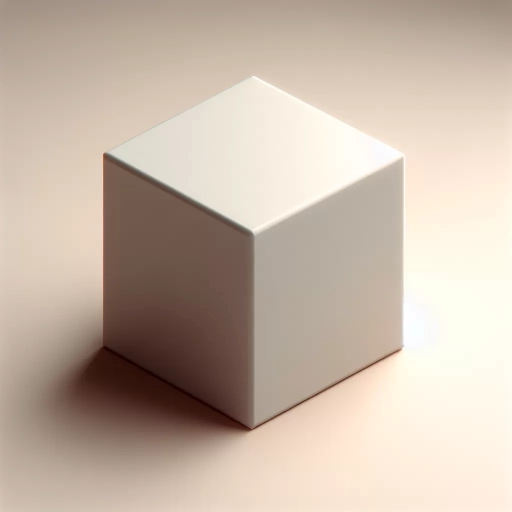
A solid structure is a fundamental concept in various fields, including engineering, architecture, and materials science. It refers to a rigid and stable form that maintains its shape under external forces, such as gravity, wind, or stress. Understanding solid structures is crucial for designing and constructing buildings, bridges, and other infrastructure that must withstand environmental conditions and loads. This article delves into the essence of solid structures by first exploring the basics of their composition and properties. It then examines the design and engineering principles that guide their construction, highlighting key considerations such as strength, durability, and stability. Finally, it showcases real-world applications and examples of solid structures in action, illustrating their importance in modern society. By grasping these foundational aspects, readers will gain a comprehensive understanding of what constitutes a solid structure and how it is essential in everyday life. To begin, let's start with **Understanding the Basics of Solid Structures**.
Understanding the Basics of Solid Structures
Understanding the basics of solid structures is crucial for engineers, architects, and anyone interested in the fundamental principles of construction and design. This article delves into three key aspects that form the backbone of solid structures: their definition and types, key components and materials, and historical development and evolution. By grasping these elements, one can appreciate the complexity and ingenuity involved in creating stable and durable structures. Starting with the definition and types of solid structures, we will explore how different classifications such as beams, columns, and arches serve specific functions and contribute to overall structural integrity. This foundational knowledge sets the stage for understanding the key components and materials that make up these structures, including metals, concrete, and wood, each with its unique properties and applications. Finally, examining the historical development and evolution of solid structures reveals how ancient civilizations to modern engineers have innovated and refined construction techniques over time. By understanding these three pillars, readers will gain a comprehensive insight into the world of solid structures. Let us begin by defining and categorizing these structures to lay a solid foundation for our exploration.
Definition and Types of Solid Structures
Solid structures are fundamental components in various fields such as engineering, architecture, and materials science. At its core, a solid structure refers to a three-dimensional arrangement of atoms, molecules, or ions that are tightly packed and have a fixed position in space. This definition encompasses a wide range of materials, from metals and ceramics to polymers and composites. There are several key types of solid structures, each characterized by the arrangement and bonding of its constituent particles. **Crystalline solids** are perhaps the most well-known, where atoms, molecules, or ions are arranged in a repeating pattern known as a crystal lattice. This regular arrangement gives crystalline solids their unique properties, such as high strength and thermal conductivity. Examples include metals like iron and copper, as well as minerals like quartz. In contrast, **amorphous solids** lack a long-range order in their atomic or molecular arrangement. Instead, these particles are randomly distributed, leading to properties that are often different from those of crystalline solids. Glass is a classic example of an amorphous solid; it does not have a crystalline structure but still exhibits rigidity and solidity. Another important category is **polycrystalline solids**, which consist of multiple crystalline regions or grains that are randomly oriented with respect to each other. These grains are separated by grain boundaries, which can affect the mechanical properties of the material. Many metals and alloys used in engineering applications are polycrystalline. **Molecular solids** are formed by weak intermolecular forces such as van der Waals forces or hydrogen bonds between molecules. These solids typically have lower melting points compared to ionic or covalent solids because the forces holding them together are weaker. Ice and sugar are examples of molecular solids. **Ionic solids** are composed of ions held together by electrostatic forces. These solids are usually hard and brittle with high melting points due to the strong ionic bonds. Common examples include sodium chloride (table salt) and calcium carbonate (limestone). **Covalent solids**, also known as network solids, consist of atoms covalently bonded to each other in a continuous three-dimensional network. These solids are extremely hard and have very high melting points due to the strong covalent bonds. Diamond and silicon carbide are notable examples. Understanding these different types of solid structures is crucial for designing and engineering materials with specific properties for various applications. Each type of solid structure has its unique characteristics that make it suitable for different uses, from construction materials to electronic components. By recognizing the underlying structure of a solid, scientists and engineers can tailor materials to meet specific requirements, enhancing performance and efficiency across a broad spectrum of industries.
Key Components and Materials
When understanding the basics of solid structures, it is crucial to delve into the key components and materials that constitute these structures. Solid structures, whether they are buildings, bridges, or any other type of construction, rely on several fundamental elements to ensure stability, durability, and functionality. **Key Components:** 1. **Foundation:** The foundation is the base of any solid structure, transferring loads from the building to the ground. It must be designed to withstand various forces such as weight, wind, and seismic activity. 2. **Frames:** The frame provides the structural skeleton of the building, supporting walls, floors, and roofs. Frames can be made from materials like steel, wood, or concrete. 3. **Walls:** Walls serve multiple purposes including load-bearing support, insulation, and protection from external elements. They can be constructed from materials like brick, concrete blocks, or wood. 4. **Roofs:** Roofs protect the structure from weather conditions and can be designed in various forms such as flat, pitched, or domed. 5. **Floors:** Floors provide a surface for occupancy and can be made from materials like concrete, wood, or composite materials. **Materials:** 1. **Concrete:** A composite material made from cement, water, and aggregate (sand or gravel), concrete is widely used for foundations, walls, and floors due to its strength and durability. 2. **Steel:** Known for its high tensile strength and versatility, steel is often used in framing structures such as beams and columns. 3. **Wood:** Wood is a natural material that has been used historically for framing and other structural elements due to its strength-to-weight ratio and ease of use. 4. **Bricks:** Bricks are made from clay and shale; they are durable and resistant to weathering but require mortar for bonding. 5. **Composites:** Composite materials like fiber-reinforced polymers (FRP) combine different materials to achieve enhanced properties such as strength and resistance to corrosion. **Additional Considerations:** - **Reinforcement:** In many cases, especially with concrete structures, reinforcement with rebar (reinforcing bars) is necessary to enhance tensile strength. - **Insulation:** Proper insulation is critical for energy efficiency and comfort within the structure. - **Sustainability:** Modern construction often considers sustainable materials that minimize environmental impact while maintaining structural integrity. Understanding these key components and materials is essential for designing and constructing solid structures that are safe, efficient, and long-lasting. Each component plays a vital role in ensuring the overall stability and functionality of the structure. By selecting appropriate materials based on their properties and intended use within the structure, engineers and architects can create robust solid structures that meet various needs while adhering to safety standards and environmental considerations.
Historical Development and Evolution
The historical development and evolution of solid structures are deeply intertwined with human civilization, reflecting advancements in technology, materials, and engineering knowledge. From ancient times to the present day, the construction of solid structures has been driven by the need for shelter, defense, and community development. In ancient civilizations such as Egypt, Greece, and Rome, solid structures were built using stone, brick, and mortar. The Egyptians developed sophisticated masonry techniques evident in the pyramids, while the Greeks and Romans perfected the use of arches and domes, as seen in structures like the Pantheon. These early innovations laid the groundwork for later architectural achievements. The Middle Ages saw the rise of Gothic architecture in Europe, characterized by tall, slender structures supported by ribbed vaults and flying buttresses. This period also witnessed significant advancements in bridge construction, with the development of stone bridges that could span longer distances. The Industrial Revolution marked a significant turning point in the evolution of solid structures. New materials like iron and steel became available, allowing for the construction of larger, more complex buildings and infrastructure projects such as railways and bridges. The introduction of reinforced concrete in the late 19th century further expanded possibilities for building design and scale. In the 20th century, advancements in materials science led to the development of new materials like prestressed concrete and composite materials. These innovations enabled the construction of high-rise buildings, long-span bridges, and other megastructures that define modern urban landscapes. The advent of computer-aided design (CAD) and finite element analysis (FEA) has also revolutionized structural engineering by allowing for precise simulations and optimizations. Today, solid structures continue to evolve with advancements in sustainable materials, green building practices, and innovative construction techniques. The integration of smart technologies and sensors into building design enhances structural integrity and efficiency. Additionally, there is a growing focus on resilience and adaptability in response to natural disasters and climate change. Understanding these historical developments is crucial for appreciating the basics of solid structures. Each era's contributions have built upon previous knowledge, leading to the sophisticated engineering practices we see today. By recognizing this evolution, engineers can better design, construct, and maintain solid structures that meet contemporary needs while respecting the lessons of history.
Design and Engineering Principles
Design and engineering principles are the cornerstone of creating robust, efficient, and safe structures. These principles encompass a wide range of critical aspects, each contributing to the overall quality and functionality of a design. Firstly, **Load Distribution and Stress Analysis** is essential for understanding how forces act on a structure, ensuring that it can withstand various types of stress without failure. Secondly, **Structural Integrity and Stability** focuses on maintaining the stability and durability of the structure over time, preventing collapse or deformation. Lastly, **Modern Design Tools and Software** enable engineers to simulate, analyze, and optimize designs with unprecedented precision, streamlining the development process. By integrating these elements, engineers can create structures that are not only aesthetically pleasing but also highly functional and safe. Understanding how loads are distributed and stresses are analyzed is crucial for laying the foundation of any successful engineering project, which is why we will first delve into the intricacies of **Load Distribution and Stress Analysis**.
Load Distribution and Stress Analysis
Load distribution and stress analysis are crucial components of design and engineering principles, particularly when it comes to ensuring the integrity and longevity of solid structures. These analyses involve determining how external forces are distributed across a structure and evaluating the resulting stresses to prevent failure. Load distribution considers various types of loads such as dead loads (the weight of the structure itself), live loads (temporary loads like people or furniture), wind loads, seismic loads, and other environmental factors. By accurately distributing these loads, engineers can identify areas of high stress concentration and design the structure to withstand these forces without compromising its stability. Stress analysis, often conducted using finite element methods or classical beam theory, helps engineers quantify the internal forces within a structure. This involves calculating tensile, compressive, shear, and torsional stresses that arise from different loading conditions. The goal is to ensure that the material's strength exceeds the maximum stress experienced at any point in the structure. Material properties such as Young's modulus, Poisson's ratio, and yield strength are critical in this analysis. Advanced computational tools and software enable detailed simulations that can predict how a structure will behave under various scenarios, allowing for iterative design improvements. Effective load distribution and stress analysis also consider factors like structural redundancy, which ensures that if one component fails, others can take over its load-bearing role. Additionally, engineers must account for dynamic loads that change over time and potential defects or imperfections in materials. By integrating these considerations into the design process, engineers can create robust structures that are both safe and efficient. This holistic approach not only enhances structural reliability but also optimizes material usage and minimizes construction costs. Ultimately, thorough load distribution and stress analysis are essential for ensuring that solid structures meet their intended performance criteria and stand the test of time.
Structural Integrity and Stability
Structural integrity and stability are fundamental principles in design and engineering, ensuring that a structure can withstand various loads and stresses without failing. This involves a comprehensive understanding of the material properties, geometric configuration, and the dynamic interactions between the structure and its environment. Engineers employ several key strategies to achieve structural integrity: first, they select materials with appropriate strength, stiffness, and durability to meet the anticipated loads. Second, they design the structure's geometry to optimize load distribution, often using techniques such as triangulation to enhance stability. Third, they apply principles of statics and dynamics to analyze how the structure will behave under different types of loads, including dead loads (the weight of the structure itself), live loads (temporary loads such as people or vehicles), wind loads, seismic loads, and other external forces. Advanced computational methods like finite element analysis (FEA) are also utilized to simulate real-world conditions and predict potential failure points. Additionally, engineers must consider factors such as fatigue, corrosion, and thermal expansion to ensure long-term stability. Regular inspections and maintenance are crucial for detecting any signs of degradation or damage that could compromise the structure's integrity. Compliance with building codes and standards further ensures that structures meet minimum safety requirements. By integrating these elements—material selection, geometric design, load analysis, computational modeling, and ongoing maintenance—engineers can create structures that are both safe and durable, thereby upholding the critical principles of structural integrity and stability in design and engineering. This holistic approach not only safeguards human life but also protects investments by extending the lifespan of structures while minimizing the need for costly repairs or replacements.
Modern Design Tools and Software
Modern design tools and software have revolutionized the field of design and engineering, significantly enhancing the efficiency, accuracy, and creativity of the design process. At the heart of these advancements are Computer-Aided Design (CAD) systems, which allow designers to create detailed 2D and 3D models with precision. Software like Autodesk AutoCAD, SolidWorks, and Fusion 360 provide robust platforms for drafting, modeling, and simulating complex structures. These tools enable designers to visualize and analyze their designs in real-time, making it easier to identify potential flaws and optimize structural integrity. Another crucial aspect is Building Information Modeling (BIM) software, such as Revit and ArchiCAD, which integrates architectural, engineering, and construction data into a single model. BIM facilitates better collaboration among stakeholders by providing a comprehensive view of the project, including spatial relationships, quantities, and properties of building components. This holistic approach ensures that all aspects of a structure are considered from the outset, leading to more cohesive and solid structures. In addition to CAD and BIM, computational design tools like Rhino and Grasshopper have become indispensable for creating complex geometries and parametric designs. These tools leverage algorithms to generate and manipulate shapes, allowing for innovative solutions that would be impossible to achieve manually. They are particularly useful in fields such as architecture and product design where unique forms can provide functional advantages. Finite Element Analysis (FEA) software, such as ANSYS and Abaqus, plays a critical role in ensuring the structural integrity of designs. FEA allows engineers to simulate various loads and stresses on a structure, predicting how it will behave under different conditions. This capability is essential for validating design assumptions and making necessary adjustments before physical prototypes are built. Furthermore, 3D printing and additive manufacturing technologies have transformed the prototyping phase by enabling rapid creation of physical models directly from digital designs. This accelerates the feedback loop between design iterations, allowing for quicker refinement and optimization of structural elements. Collaboration tools such as Slack, Trello, and Asana also play a vital role in modern design processes by facilitating communication among team members. These platforms ensure that all stakeholders are aligned with project goals and timelines, reducing misunderstandings that could compromise structural integrity. In conclusion, modern design tools and software are integral to the principles of solid structure design. They enhance precision, foster collaboration, and enable the creation of complex yet robust structures. By leveraging these technologies effectively, designers and engineers can ensure that their creations are not only aesthetically pleasing but also structurally sound.
Applications and Real-World Examples
The applications and real-world examples of advanced materials and technologies are vast and transformative, impacting various sectors in profound ways. From the iconic architectural landmarks and buildings that redefine urban skylines, to the intricate infrastructure projects such as bridges and roads that connect communities, these innovations are pivotal. Additionally, they play a crucial role in industrial and residential uses, enhancing efficiency, sustainability, and quality of life. Architectural landmarks, for instance, leverage cutting-edge materials to achieve unprecedented structural integrity and aesthetic appeal. The use of high-strength concrete, advanced steel alloys, and innovative glass technologies allows architects to push the boundaries of design. These advancements not only create visually stunning structures but also ensure they are durable and environmentally friendly. Transitioning to the realm of architectural landmarks and buildings, we see how these technologies are revolutionizing the way we construct and interact with our built environment. --- **Architectural Landmarks and Buildings**
Architectural Landmarks and Buildings
Architectural landmarks and buildings are not just physical structures; they are testaments to human ingenuity, cultural expression, and historical significance. These solid structures serve as more than mere shelters; they embody the essence of their time and place, reflecting societal values, technological advancements, and artistic visions. For instance, the Eiffel Tower in Paris, built for the 1889 World's Fair, exemplifies late 19th-century engineering prowess and has become an iconic symbol of French culture. Similarly, the Taj Mahal in India, constructed by Mughal Emperor Shah Jahan in the 17th century, is a masterpiece of Mughal architecture that blends Indian, Persian, and Islamic styles, showcasing the rich cultural heritage of the region. These structures also play a crucial role in urban planning and community development. The Guggenheim Museum in Bilbao, Spain, designed by Frank Gehry, is a prime example of how a single building can transform a city's landscape. Since its opening in 1997, it has become a catalyst for urban renewal, attracting millions of visitors and stimulating local economic growth. In addition to their aesthetic appeal, many architectural landmarks are designed with sustainability in mind. The Sydney Opera House, with its distinctive sail-like design, incorporates innovative materials and energy-efficient systems that minimize its environmental footprint. From a functional perspective, these buildings often serve multiple purposes beyond their primary use. The Burj Khalifa in Dubai, currently the world's tallest building, is not only a residential and commercial hub but also a tourist attraction and a symbol of modern engineering. It features advanced systems for water conservation and energy efficiency, making it a model for sustainable urban development. Furthermore, historical landmarks like the Colosseum in Rome or the Great Wall of China are preserved and protected as part of cultural heritage initiatives, providing valuable insights into past civilizations and contributing to educational and tourism sectors. In terms of real-world applications, architectural landmarks influence various fields such as architecture, engineering, urban planning, and even psychology. For example, studies on the psychological impact of built environments have shown that well-designed public spaces can enhance community cohesion and mental well-being. The design principles behind iconic buildings like the Louvre Pyramid in Paris or the Chrysler Building in New York City have inspired countless other projects around the world, demonstrating how architectural innovation can be replicated and adapted across different contexts. Moreover, these structures often become integral parts of national identity and pride. The White House in Washington D.C., for instance, is not just the official residence of the U.S. President but also a symbol of American democracy and history. Similarly, the Acropolis of Athens stands as a testament to ancient Greek civilization and continues to inspire architectural designs globally. In conclusion, architectural landmarks and buildings are more than just solid structures; they are living embodiments of human creativity, technological advancement, and cultural richness. They serve as educational tools, economic drivers, and symbols of national identity, making them indispensable components of our built environment. Their impact extends beyond their physical presence to influence various aspects of society, from urban planning and sustainability to cultural heritage and psychological well-being.
Infrastructure Projects: Bridges and Roads
Infrastructure projects, particularly bridges and roads, are crucial components of a solid structure that underpin modern society. These projects not only facilitate transportation but also drive economic growth, enhance safety, and improve quality of life. Bridges, for instance, connect disparate regions, enabling the efficient movement of goods and people. The Golden Gate Bridge in San Francisco is a prime example; it has been a vital artery for traffic since its completion in 1937, significantly reducing travel time between the city and Marin County. Similarly, the Akashi Kaikyo Bridge in Japan, known for its impressive span of over 3.9 kilometers, exemplifies engineering excellence and has become a symbol of technological advancement. Roads are equally essential, forming the backbone of transportation networks. Highways like the Interstate Highway System in the United States have revolutionized long-distance travel and commerce. The Autobahn in Germany, famous for its high-speed limits, demonstrates how well-designed road infrastructure can support both economic development and public safety. In developing countries, road construction projects often serve as catalysts for rural development by providing access to markets, healthcare facilities, and educational institutions. The construction of bridges and roads involves meticulous planning, advanced engineering techniques, and robust materials to ensure durability and safety. For example, the use of high-strength concrete and advanced steel alloys has allowed for the creation of longer-lasting structures that can withstand harsh environmental conditions. Additionally, innovative technologies such as smart traffic management systems and intelligent transportation systems (ITS) are being integrated into these projects to enhance efficiency and reduce congestion. Real-world examples abound where infrastructure projects have transformed communities. The construction of the M4 Motorway in the UK, for instance, has significantly reduced travel times between major cities like London and Bristol, boosting regional economic activity. In Australia, the WestConnex project aims to alleviate traffic congestion in Sydney by creating new motorway connections, thereby improving air quality and reducing commute times. Moreover, sustainable practices are increasingly being incorporated into these projects. Green infrastructure initiatives focus on minimizing environmental impact through the use of recycled materials, energy-efficient lighting systems, and eco-friendly construction methods. The Netherlands' innovative approach to flood-resistant road design is another example where infrastructure is adapted to mitigate the effects of climate change. In conclusion, bridges and roads are fundamental to a solid structure that supports modern civilization. These infrastructure projects not only facilitate mobility but also contribute to economic prosperity, public safety, and environmental sustainability. As technology continues to evolve, future infrastructure projects will likely incorporate even more advanced materials and intelligent systems to meet the demands of a rapidly changing world.
Industrial and Residential Uses
In the realm of solid structures, both industrial and residential applications are pivotal, showcasing the versatility and necessity of these constructs in everyday life. **Industrial uses** of solid structures are multifaceted and critical for operational efficiency. For instance, warehouses and manufacturing facilities rely on robust solid structures to support heavy machinery, storage systems, and production lines. These structures ensure stability and safety, allowing for the smooth operation of industrial processes. Additionally, bridges and tunnels, which are quintessential examples of solid structures, facilitate transportation networks that are essential for the movement of goods and people. In the energy sector, solid structures are used in the construction of power plants, wind turbines, and solar panels, ensuring the reliable generation and distribution of energy. Furthermore, industrial buildings such as factories and refineries utilize solid structures to withstand extreme conditions like high temperatures and pressures, thereby safeguarding both the equipment and personnel. **Residential uses** of solid structures are equally important as they provide the foundation for safe and comfortable living spaces. Homes, apartments, and condominiums are all built using solid structures that ensure structural integrity and durability. These structures protect inhabitants from environmental elements such as wind, rain, and extreme temperatures while also providing a stable environment that supports daily activities. In urban planning, solid structures are integral in the development of high-rise buildings, which maximize land use in densely populated areas. Moreover, community facilities like schools, hospitals, and libraries depend on solid structures to create secure and functional spaces that serve the needs of the community. The use of solid structures in residential construction also allows for innovative architectural designs that balance aesthetics with functionality, enhancing the quality of life for residents. In both industrial and residential contexts, the application of solid structures is not just about functionality but also about sustainability and environmental responsibility. Modern solid structures often incorporate green building materials and techniques that reduce environmental impact while maintaining structural integrity. This dual focus on performance and sustainability underscores the evolving role of solid structures in meeting contemporary needs while ensuring a resilient future. By understanding the diverse applications of solid structures across different sectors, we can appreciate their fundamental importance in shaping our built environment and supporting various aspects of human activity.