What Is The Major Product For The Following Reaction?
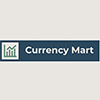
In the realm of organic chemistry, understanding the outcomes of chemical reactions is crucial for synthesizing desired compounds. One such reaction, which has garnered significant attention due to its complexity and importance, involves the transformation of a specific substrate into various products. The major product of this reaction is a key focus, as it determines the efficacy and direction of the synthesis process. To delve into this topic, it is essential to break down the analysis into three critical components: **Understanding the Reaction Mechanism**, **Identifying Major Products**, and **Experimental Verification and Analysis**. By grasping the underlying mechanism, chemists can predict and control the reaction pathway. Identifying the major products helps in optimizing reaction conditions to favor the desired outcome. Finally, experimental verification ensures that theoretical predictions align with practical results. This article will explore these aspects in detail, starting with a thorough examination of **Understanding the Reaction Mechanism**.
Understanding the Reaction Mechanism
Understanding the reaction mechanism is a crucial aspect of chemistry, as it provides insights into how chemical reactions proceed and how they can be optimized. This comprehensive understanding is built on three key pillars: identifying key reactants and catalysts, analyzing reaction conditions, and determining reaction pathways. By identifying the key reactants and catalysts involved in a reaction, chemists can pinpoint the molecular entities that drive the transformation, allowing for precise control over the reaction's outcome. Analyzing reaction conditions such as temperature, pressure, and solvent composition helps in optimizing the reaction environment to achieve desired results. Finally, determining the reaction pathways elucidates the step-by-step process by which reactants are converted into products, revealing potential bottlenecks and opportunities for improvement. Each of these components is essential for a thorough grasp of the reaction mechanism. To begin this exploration, it is critical to start by identifying key reactants and catalysts, as these are the foundational elements that set the stage for the entire reaction process.
Identifying Key Reactants and Catalysts
Identifying key reactants and catalysts is a crucial step in understanding the reaction mechanism, as it allows chemists to predict the major product of a chemical reaction. Reactants are the substances that undergo chemical change to form products, while catalysts are substances that speed up the reaction without being consumed or altered in the process. To identify these components, one must carefully analyze the reaction equation and consider the roles of each molecule involved. In a typical reaction, reactants are listed on the left side of the arrow, and products are listed on the right. Catalysts, if present, are often indicated above or below the arrow. For example, in the reaction \( \text{A} + \text{B} \xrightarrow{\text{Catalyst}} \text{Product} \), A and B are reactants, and Catalyst is speeding up the reaction. Understanding which molecules are reactants and which are catalysts helps in determining the reaction pathway and identifying potential intermediates. Moreover, recognizing key reactants involves identifying their functional groups and how these groups interact with other molecules. Functional groups are specific groups of atoms within a molecule that determine its chemical properties and reactivity. For instance, in an organic reaction involving an alcohol (R-OH) and an acid (R-COOH), the hydroxyl (-OH) group of the alcohol and the carboxyl (-COOH) group of the acid are critical for understanding how these molecules will react. Catalysts can be either homogeneous (dissolved in the reaction mixture) or heterogeneous (in a different phase than the reactants). Identifying whether a catalyst is homogeneous or heterogeneous is important because it affects how the catalyst interacts with reactants and influences the reaction rate. For example, in hydrogenation reactions, palladium (Pd) is often used as a heterogeneous catalyst to facilitate the addition of hydrogen to unsaturated compounds. By accurately identifying key reactants and catalysts, chemists can apply mechanistic principles such as nucleophilic substitution, elimination reactions, or addition reactions to predict the major product. This involves considering factors like steric effects, electronic effects, and thermodynamic stability to determine which pathway is most favorable. In summary, identifying key reactants and catalysts is essential for understanding the reaction mechanism and predicting the major product of a chemical reaction. This involves analyzing the reaction equation, recognizing functional groups in reactants, distinguishing between homogeneous and heterogeneous catalysts, and applying mechanistic principles to determine the most likely reaction pathway. By doing so, chemists can better control and optimize chemical reactions in various fields such as organic synthesis, industrial processes, and pharmaceutical development.
Analyzing Reaction Conditions
Analyzing reaction conditions is a crucial step in understanding the reaction mechanism and predicting the major product of a chemical reaction. This involves examining various factors such as temperature, pressure, solvent, catalysts, and the concentration of reactants. Temperature, for instance, can significantly influence the rate and outcome of a reaction by altering the kinetic energy of the molecules and potentially changing the reaction pathway. Higher temperatures often increase the reaction rate but may also favor side reactions or decomposition, while lower temperatures can lead to more selective reactions. Pressure is another critical factor, particularly in gas-phase reactions where it affects the concentration of reactants. Increased pressure can drive equilibrium reactions towards the side with fewer moles of gas, according to Le Chatelier's principle. The choice of solvent is equally important as it can influence the solubility of reactants and products, stabilize transition states, or even participate in the reaction mechanism. Polar solvents, for example, are often used in reactions involving charged intermediates to stabilize them. Catalysts play a pivotal role by lowering the activation energy required for the reaction to proceed, thereby increasing the reaction rate without being consumed in the process. They can be homogeneous (dissolved in the solvent) or heterogeneous (solid catalysts). The concentration of reactants also impacts the reaction outcome; higher concentrations typically increase the reaction rate but may lead to unwanted side reactions if not controlled properly. Understanding these reaction conditions allows chemists to optimize the reaction setup to favor the formation of the desired product. For instance, in a Diels-Alder reaction, which is a [4+2] cycloaddition between a diene and a dienophile, controlling temperature and pressure can help in achieving high yields of the cycloadduct. Similarly, in an aldol condensation, the choice of base and solvent can determine whether the reaction proceeds to form the aldol product or further dehydrates to form an enone. By meticulously analyzing and adjusting these reaction conditions, chemists can gain insights into the reaction mechanism and predict with greater accuracy which product will be formed in higher yield. This systematic approach not only enhances the efficiency of chemical synthesis but also aids in understanding the underlying principles that govern chemical transformations, ultimately leading to better control over the reaction outcome and higher yields of the desired product.
Determining Reaction Pathways
Determining reaction pathways is a crucial step in understanding the reaction mechanism, as it helps chemists predict the major product of a chemical reaction. This process involves identifying the sequence of steps through which reactants are converted into products. To determine these pathways, chemists often use various analytical techniques and theoretical models. For instance, kinetic studies can provide insights into the rate-determining step, while spectroscopic methods such as NMR and IR spectroscopy can help identify intermediate species. Computational chemistry tools, like density functional theory (DFT) and molecular mechanics, are also employed to simulate potential energy surfaces and predict the most favorable reaction routes. Additionally, the Hammond-Leffler postulate and the Curtin-Hammett principle are theoretical frameworks that aid in understanding the relationship between transition states and intermediates, thereby guiding the identification of the most likely reaction pathway. By combining these approaches, chemists can construct a detailed mechanism that explains how reactants transform into products, ultimately allowing them to predict the major product of a given reaction with high accuracy. This comprehensive understanding is essential for optimizing reaction conditions, improving yields, and designing new synthetic routes in organic and inorganic chemistry.
Identifying Major Products
Identifying major products in chemical reactions is a critical skill that requires a comprehensive understanding of several key factors. To accurately determine the primary products, one must first recognize the patterns of product formation, which involves understanding the mechanisms and conditions under which reactions occur. This knowledge helps in predicting the likely outcomes of a reaction. Additionally, evaluating the stability and yield of potential products is essential, as it allows chemists to assess the feasibility and efficiency of the reaction. Finally, comparing possible product structures helps in distinguishing between plausible and less likely outcomes, ensuring that the correct product is identified. By integrating these approaches—recognizing product formation patterns, evaluating product stability and yield, and comparing possible product structures—chemists can confidently identify the major products of a reaction. Let's begin by examining the first of these crucial steps: recognizing product formation patterns.
Recognizing Product Formation Patterns
Recognizing product formation patterns is a crucial skill in organic chemistry, particularly when identifying the major products of chemical reactions. This involves understanding the mechanisms and reactivity of different functional groups, as well as the conditions under which reactions occur. For instance, in nucleophilic substitution reactions, the major product can often be predicted by considering the leaving group ability and the nucleophile's strength. In SN1 reactions, the formation of a carbocation intermediate leads to racemization and potential rearrangements, while SN2 reactions proceed with inversion of configuration and no rearrangement. In elimination reactions, such as E1 and E2, the major product depends on the substrate's structure and reaction conditions. E1 reactions typically yield the more stable alkene due to carbocation formation, whereas E2 reactions favor the more substituted alkene due to transition state stability. In addition, understanding the concept of regioselectivity, as seen in Markovnikov's rule for electrophilic addition to alkenes, helps predict the major product by identifying the more stable carbocation intermediate. Furthermore, recognizing patterns in pericyclic reactions like Diels-Alder and Claisen rearrangements involves understanding orbital interactions and symmetry rules. These reactions often lead to specific stereochemical outcomes that can be predicted based on the reactants' structures. In aromatic substitution reactions, the directing effects of substituents on the benzene ring determine the position of the incoming electrophile. For example, ortho/para directors like methyl and hydroxyl groups lead to different major products compared to meta directors like nitro and carboxyl groups. Lastly, recognizing product formation patterns also involves considering steric effects and thermodynamic stability. For example, in aldol condensations, the major product is often influenced by steric factors that favor the formation of less hindered enolates. By mastering these patterns and principles, chemists can accurately predict and identify the major products of various organic reactions, which is essential for synthesizing complex molecules and understanding reaction mechanisms. This skill is fundamental in both academic research and industrial applications where precise control over reaction outcomes is critical.
Evaluating Product Stability and Yield
When evaluating product stability and yield in chemical reactions, several key factors must be considered to ensure accurate and reliable results. **Product Stability** is crucial as it determines the longevity and integrity of the formed compounds. This involves assessing the chemical structure of the product for potential instability due to factors such as steric strain, electronic instability, or susceptibility to degradation under various conditions like heat, light, or moisture. For instance, in reactions involving highly reactive intermediates or sensitive functional groups, special care must be taken to stabilize these products through appropriate handling and storage techniques. **Yield**, on the other hand, refers to the amount of product obtained relative to the theoretical maximum amount that could be produced based on the limiting reagent. High yield indicates efficient reaction conditions and minimal side reactions or losses during workup and purification. To optimize yield, chemists often focus on reaction conditions such as temperature, solvent choice, catalysts, and reaction time. For example, in a synthesis involving multiple steps, each step's yield can significantly impact the overall yield of the final product; thus, optimizing each step individually is essential. In addition to these factors, **analytical techniques** play a vital role in evaluating both stability and yield. Methods like Nuclear Magnetic Resonance (NMR) spectroscopy, Mass Spectrometry (MS), and High-Performance Liquid Chromatography (HPLC) are used to confirm the structure and purity of the product. These techniques help identify any impurities or by-products that might affect stability and overall yield. Moreover, understanding **kinetics and thermodynamics** of the reaction can provide insights into how to maximize yield and ensure product stability. Kinetic studies can reveal the rate-determining step in a reaction pathway, allowing for optimization of conditions to favor the desired product. Thermodynamic considerations help in predicting whether a reaction is spontaneous under given conditions and if there are any equilibrium limitations that need to be addressed. Finally, **scalability** is an important consideration when evaluating product stability and yield. Reactions that are efficient on a small scale may not translate well to larger scales due to differences in heat transfer, mixing efficiency, or other factors. Therefore, pilot-scale experiments are often conducted to ensure that the optimized conditions remain effective when scaled up. By carefully evaluating these aspects—product stability, yield optimization through reaction conditions and analytical techniques, understanding kinetics and thermodynamics, and considering scalability—chemists can effectively identify major products in chemical reactions with high accuracy and reliability. This comprehensive approach ensures that the identified major product is not only the primary outcome but also stable and obtainable in significant quantities.
Comparing Possible Product Structures
When identifying the major product of a chemical reaction, it is crucial to compare possible product structures to determine which one is most likely to form. This involves analyzing the reaction mechanism, considering factors such as thermodynamic stability, kinetic favorability, and steric effects. For instance, in an elimination reaction, the major product can be predicted by comparing the stability of possible alkenes formed. The more stable alkene, typically the one with more substituted double bonds (Zaitsev's rule), is usually the major product. Similarly, in a nucleophilic substitution reaction, the major product can depend on whether the reaction follows an SN1 or SN2 pathway. In an SN1 reaction, the carbocation intermediate can lead to rearrangements or the formation of a more stable carbocation, influencing the final product. In contrast, SN2 reactions are more stereospecific and less likely to result in rearrangements. Additionally, steric hindrance plays a significant role; for example, in reactions involving bulky groups, the less hindered pathway often leads to the major product. By carefully evaluating these structural factors and reaction conditions, chemists can accurately predict and identify the major product of a given reaction. This systematic approach ensures that all possible outcomes are considered, leading to a more comprehensive understanding of the reaction's outcome and facilitating the synthesis of desired compounds with higher efficiency and selectivity.
Experimental Verification and Analysis
Experimental verification and analysis are crucial components in scientific research, ensuring that hypotheses are rigorously tested and results are accurately interpreted. This process involves several key steps that collectively contribute to the validity and reliability of experimental findings. First, **conducting laboratory experiments** is essential as it allows researchers to manipulate variables and observe their effects under controlled conditions. Second, **using spectroscopic techniques for identification** enables the precise characterization of substances, which is vital for understanding chemical structures and reactions. Third, **quantifying product yields and purity** helps in assessing the efficiency and effectiveness of experimental procedures. By integrating these methodologies, scientists can gather comprehensive data, validate their results, and draw meaningful conclusions. This article will delve into each of these aspects, beginning with the foundational importance of conducting laboratory experiments.
Conducting Laboratory Experiments
Conducting laboratory experiments is a crucial step in the scientific process, particularly when it comes to experimental verification and analysis. These experiments are designed to test hypotheses, validate theories, and gather data that can be analyzed to draw meaningful conclusions. When conducting laboratory experiments, it is essential to follow a structured approach to ensure accuracy, reliability, and reproducibility of the results. First, a clear hypothesis or research question must be defined. This guides the entire experiment and helps in designing the methodology. The next step involves selecting appropriate materials and equipment, ensuring they are calibrated and in good working condition. A detailed protocol outlining each step of the experiment should be developed, including any necessary safety precautions. During the experiment, meticulous attention to detail is vital. Measurements should be taken accurately, and data should be recorded systematically. It is also important to control for variables that could affect the outcome, using techniques such as randomization and blinding where applicable. Replication of the experiment multiple times helps in establishing the reliability of the findings. After collecting data, it must be analyzed using appropriate statistical methods to identify trends, patterns, and correlations. This analysis should be thorough yet unbiased, considering both expected and unexpected results. The findings should then be compared with existing literature to see if they align with or contradict previous studies. Finally, the results of the experiment should be documented clearly in a report or paper. This includes describing the methodology used, presenting the data collected, discussing the implications of the findings, and drawing conclusions based on the analysis. Proper citation of sources and adherence to ethical standards are also crucial. In the context of determining the major product for a given reaction, laboratory experiments play a pivotal role. For instance, if we are investigating a chemical reaction where multiple products are possible, experiments can help identify which product is formed in greater quantities under specific conditions. Techniques such as chromatography, spectroscopy, or other analytical methods can be employed to isolate and characterize the products. By following these steps rigorously, scientists can ensure that their experiments are robust and their conclusions are well-supported by evidence. This not only enhances the credibility of their research but also contributes significantly to the advancement of scientific knowledge. In summary, conducting laboratory experiments is an indispensable part of experimental verification and analysis, allowing researchers to validate hypotheses and uncover new insights with precision and reliability.
Using Spectroscopic Techniques for Identification
In the realm of chemical analysis, spectroscopic techniques play a pivotal role in the identification of compounds, particularly when verifying the major product of a reaction. These methods leverage the interaction between matter and electromagnetic radiation to provide detailed information about molecular structure and composition. For instance, Nuclear Magnetic Resonance (NMR) spectroscopy is widely used to determine the structure of organic compounds by analyzing the magnetic properties of nuclei. By interpreting the NMR spectrum, chemists can identify the types of hydrogen and carbon atoms present in a molecule, their relative positions, and even the presence of functional groups. Infrared (IR) spectroscopy is another powerful tool that helps in identifying functional groups within a molecule. IR spectra show absorption peaks corresponding to specific vibrational modes of bonds, allowing researchers to confirm the presence or absence of particular functional groups such as hydroxyl (-OH), carbonyl (C=O), or amine (-NH2) groups. This is crucial for verifying the formation of expected products in a reaction. Mass Spectrometry (MS) provides complementary information by measuring the mass-to-charge ratio of ions produced from the fragmentation of molecules. This technique is invaluable for determining the molecular weight and empirical formula of a compound, which can be compared with theoretical values to confirm the identity of the reaction product. Additionally, Ultraviolet-Visible (UV-Vis) spectroscopy can be used to identify compounds based on their electronic transitions. By analyzing the absorption spectrum, researchers can infer the presence of conjugated systems or specific chromophores that are indicative of certain molecular structures. The integration of these spectroscopic techniques ensures comprehensive characterization and unambiguous identification of reaction products. For example, if a reaction is expected to produce a compound with a specific functional group, IR spectroscopy can confirm its presence while NMR can provide detailed structural information. Meanwhile, MS can verify the molecular weight and empirical formula, and UV-Vis spectroscopy can offer insights into electronic transitions. In experimental verification and analysis, these spectroscopic methods are indispensable for confirming that the synthesized product matches the expected outcome. By combining data from multiple spectroscopic techniques, researchers can build a robust case for the identity of the major product, thereby validating their experimental results and ensuring the accuracy of their conclusions. This multi-faceted approach not only enhances the reliability of chemical synthesis but also facilitates deeper understanding and characterization of complex molecules.
Quantifying Product Yields and Purity
Quantifying product yields and purity is a crucial step in the experimental verification and analysis of chemical reactions. This process involves determining the amount of the desired product obtained from a reaction and assessing its purity to ensure that it meets the required standards. To quantify product yields, chemists typically use techniques such as gravimetry, where the mass of the product is measured, or chromatography, which separates and quantifies the components of a mixture based on their interactions with a stationary phase and a mobile phase. High-performance liquid chromatography (HPLC) and gas chromatography (GC) are commonly employed for this purpose due to their high sensitivity and resolution. Purity assessment often involves spectroscopic methods like nuclear magnetic resonance (NMR) spectroscopy, infrared (IR) spectroscopy, and mass spectrometry (MS). NMR spectroscopy provides detailed information about the molecular structure by analyzing the magnetic properties of atomic nuclei, while IR spectroscopy identifies functional groups within the molecule based on their vibrational frequencies. Mass spectrometry determines the molecular weight and fragmentation patterns of the compound, helping to confirm its identity and purity. In addition to these analytical techniques, chemists may also use physical methods such as melting point determination and recrystallization to further purify and characterize the product. The melting point of a compound can serve as a fingerprint for its identity, and recrystallization can significantly enhance purity by selectively precipitating the desired product from a solution. For the specific reaction in question, identifying the major product involves analyzing the reaction mechanism and stoichiometry. This typically requires understanding the reactivity of the reactants, potential side reactions, and any catalysts or conditions that might influence the outcome. By combining data from yield quantification with purity analysis, researchers can confidently determine whether the reaction has produced the desired major product in sufficient quantity and quality. In summary, quantifying product yields and purity is essential for verifying the success of a chemical reaction. Through a combination of gravimetric measurements, chromatographic separations, spectroscopic analyses, and physical characterization methods, chemists can accurately determine both the amount and purity of the product obtained. This comprehensive approach ensures that experimental results are reliable and meaningful, ultimately contributing to a deeper understanding of chemical processes and their applications.