What Is Crystallization
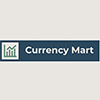
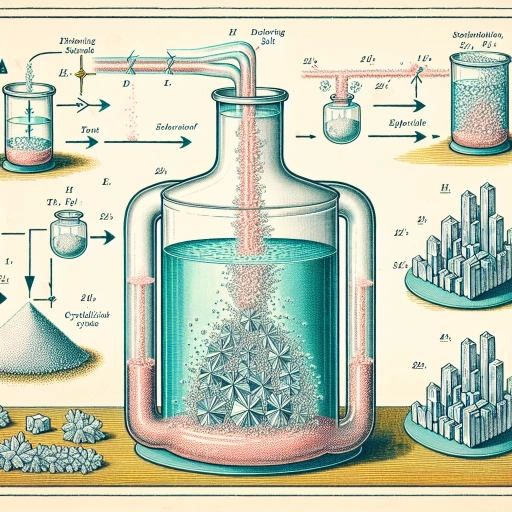
Crystallization is a fundamental process in both natural and industrial contexts, involving the formation of solid crystals from a solution, melt, or gas. This intricate phenomenon is not only aesthetically captivating but also crucial for various scientific and technological applications. To delve into the world of crystallization, it is essential to first understand its basic principles. In this article, we will explore the foundational aspects of crystallization, including the conditions and mechanisms that govern this process. We will then delve into the scientific underpinnings that explain how crystallization occurs at a molecular level. Finally, we will examine the diverse applications and techniques employed in crystallization, highlighting its significance in fields such as chemistry, pharmaceuticals, and materials science. By grasping these key elements, readers will gain a comprehensive understanding of crystallization. Let us begin by **Understanding the Basics of Crystallization**, where we will lay the groundwork for a deeper exploration of this fascinating subject.
Understanding the Basics of Crystallization
Understanding the basics of crystallization is a fundamental concept that underpins various scientific and industrial processes. Crystallization, the process by which a solid forms from a solution, melt, or gas, is not just a natural phenomenon but also a crucial technique in multiple fields. To delve into this subject, it is essential to first grasp the **Definition and Process Overview**, which lays the groundwork for understanding how crystallization occurs and the key factors influencing it. Additionally, recognizing the **Types of Crystallization** helps in identifying the specific methods and conditions required for different applications. Finally, appreciating the **Importance in Various Industries** highlights its significance in fields such as pharmaceuticals, chemistry, and materials science. By exploring these aspects, one can gain a comprehensive understanding of crystallization and its far-reaching implications. Let us begin by examining the definition and process overview of crystallization, which will provide a solid foundation for further exploration.
Definition and Process Overview
Crystallization is a fundamental process in chemistry and materials science that involves the formation of solid crystals from a solution, melt, or gas. At its core, crystallization is a phase transition where atoms, molecules, or ions arrange themselves into a repeating pattern, known as a crystal lattice. This process is crucial in various industries, including pharmaceuticals, food processing, and semiconductor manufacturing. The crystallization process can be broadly divided into several key stages. **Nucleation** is the initial step where the solution becomes supersaturated, meaning it contains more dissolved substance than it can hold at equilibrium. At this point, tiny clusters of molecules or ions begin to form, which are known as nuclei. These nuclei can either dissolve back into the solution or grow into larger crystals. **Growth** follows nucleation, where the nuclei expand as more molecules or ions adhere to their surfaces. This growth phase is highly influenced by factors such as temperature, concentration of the solution, and the presence of impurities. **Recrystallization** is another important aspect of crystallization, often used to purify substances. In this process, a solid is dissolved in a solvent and then allowed to crystallize again. This method helps in removing impurities because the crystal lattice structure tends to exclude foreign particles, resulting in a purer final product. Understanding the conditions under which crystallization occurs is vital for controlling the size, shape, and purity of the crystals. For instance, **cooling crystallization** involves slowly cooling a supersaturated solution to induce nucleation and growth. **Evaporative crystallization** involves reducing the solvent concentration through evaporation, which also leads to supersaturation and subsequent crystal formation. In addition to these methods, **seed crystals** can be introduced into the solution to facilitate nucleation. These seeds provide a template for crystal growth, helping to control the size and shape of the resulting crystals. Advanced techniques such as **sonocrystallization** and **magnetic field-induced crystallization** are also being explored for their potential to enhance crystal quality and yield. Overall, the crystallization process is a delicate balance of thermodynamic and kinetic factors. By carefully controlling these variables, scientists and engineers can produce high-quality crystals with specific properties tailored for various applications. This understanding is essential for optimizing industrial processes and ensuring the production of materials with desired characteristics.
Types of Crystallization
Crystallization, a fundamental process in various scientific and industrial fields, involves the formation of solid crystals from a solution, melt, or gas. Understanding the different types of crystallization is crucial for optimizing this process in various applications. There are several key types of crystallization, each with its own unique characteristics and uses. **1. Solution Crystallization:** This is one of the most common methods, where a solution containing the solute is cooled or concentrated to induce crystallization. The solute's concentration increases until it reaches its saturation point, at which crystals begin to form. This method is widely used in pharmaceuticals, food processing, and chemical manufacturing due to its ability to produce high-purity crystals. **2. Melt Crystallization:** In this process, a molten substance is cooled slowly to allow the formation of crystals. This technique is often employed in the production of metals and alloys, where controlled cooling rates can influence the crystal structure and properties of the final product. Melt crystallization is also used in the purification of substances like silicon for semiconductor applications. **3. Vapor Phase Crystallization:** This method involves the condensation of a vapor onto a surface, leading to the formation of crystals. It is commonly seen in natural phenomena such as frost formation but is also utilized in industrial processes like the production of nanomaterials and thin films. Vapor phase crystallization allows for precise control over crystal growth conditions. **4. Cooling Crystallization:** Cooling crystallization involves reducing the temperature of a solution or melt to induce crystallization. This can be achieved through various cooling methods, including direct cooling, indirect cooling, or evaporative cooling. The rate and method of cooling can significantly affect the size, shape, and purity of the resulting crystals. **5. Evaporative Crystallization:** In this process, the solvent is evaporated from the solution, increasing the concentration of the solute until it crystallizes. This technique is often used in the production of salts and other inorganic compounds. Evaporative crystallization can be performed under vacuum or at atmospheric pressure, depending on the specific requirements. **6. Reactive Crystallization:** Reactive crystallization occurs when a chemical reaction produces a new compound that immediately crystallizes. This method is particularly useful in situations where the reaction product is insoluble in the reaction medium, allowing for direct separation and purification of the desired compound. **7. Seeding Crystallization:** Seeding involves introducing small crystals (seeds) into a supersaturated solution to initiate crystallization. This technique helps control the size and number of crystals formed, ensuring uniformity and high purity. Seeding is crucial in biotechnology and pharmaceutical applications where consistent product quality is paramount. Each type of crystallization has its own set of advantages and challenges, and the choice of method depends on the specific requirements of the application, including the properties of the substance being crystallized, the desired purity and yield, and the available equipment and resources. Understanding these different types is essential for optimizing crystallization processes across various industries, ensuring the production of high-quality crystals that meet specific standards and specifications.
Importance in Various Industries
Crystallization is a fundamental process that holds significant importance across various industries, each leveraging its unique properties to achieve specific goals. In the pharmaceutical industry, crystallization is crucial for the production of high-purity drugs. It allows for the precise control of crystal structure and size, which can affect the solubility, stability, and bioavailability of medications. For instance, the crystalline form of a drug can influence its dissolution rate in the body, thereby impacting its efficacy and safety profile. This precision is essential for ensuring consistent therapeutic outcomes and compliance with regulatory standards. In the chemical industry, crystallization is a key step in the purification and separation of chemicals. It enables the isolation of pure compounds from complex mixtures, which is vital for producing high-quality chemicals used in manufacturing processes. For example, in the production of fertilizers, crystallization helps in separating and purifying essential nutrients like ammonium nitrate and urea, ensuring their effectiveness and minimizing environmental impact. The food industry also relies heavily on crystallization, particularly in the production of sugar and salt. The crystalline form of these commodities is essential for their texture, taste, and shelf life. Sugar crystallization, for instance, involves controlling the size and shape of sugar crystals to achieve the desired granulation, which affects the product's appearance and usability in various recipes. Similarly, salt crystallization ensures that table salt has the right texture and flow properties, making it easier to handle and store. In materials science and engineering, crystallization plays a pivotal role in the development of advanced materials with tailored properties. The process is used to create single crystals or polycrystalline structures that exhibit specific mechanical, electrical, or optical properties. For example, silicon crystals are grown through crystallization processes for use in semiconductor devices, while sapphire crystals are used in high-strength windows and optical components due to their exceptional hardness and transparency. Furthermore, in environmental science, crystallization is employed in wastewater treatment to remove contaminants such as heavy metals and salts. This process involves precipitating these impurities out of solution as crystals, which can then be easily separated and disposed of or recycled. This method is particularly effective because it allows for the recovery of valuable resources while minimizing waste and environmental pollution. In addition to these applications, crystallization has significant implications in biotechnology. It is used to purify proteins and other biological molecules, which is critical for research and development in fields like medicine and agriculture. The precise control over crystal formation enables scientists to study the structure and function of these molecules at the atomic level, leading to breakthroughs in understanding biological processes and developing new treatments. Overall, the importance of crystallization spans multiple industries, each benefiting from its ability to produce high-purity materials with specific properties. Understanding the basics of crystallization is therefore essential for advancing technological capabilities and addressing various industrial challenges effectively. By mastering this process, industries can enhance product quality, improve efficiency, and drive innovation forward.
The Science Behind Crystallization
Crystallization is a complex process that involves the transformation of a substance from a disordered state into a highly ordered crystal structure. This phenomenon is governed by several key scientific principles, each playing a crucial role in the overall crystallization process. At its core, crystallization is influenced by **Thermodynamic Principles**, which dictate the conditions under which a substance will transition from a liquid or gas to a solid state. Additionally, **Kinetic Factors and Nucleation** are essential, as they determine the rate at which crystallization occurs and the formation of the initial crystal nucleus. Finally, **Crystal Structure and Morphology** shape the final form and properties of the crystal, influencing its appearance and functionality. Understanding these interrelated aspects is vital for optimizing crystallization processes in various fields, from pharmaceuticals to materials science. By delving into these principles, we can better control and predict the outcomes of crystallization, starting with the foundational **Thermodynamic Principles** that set the stage for this intricate process.
Thermodynamic Principles
Thermodynamic principles play a pivotal role in understanding the science behind crystallization, as they govern the conditions under which crystals form and grow. At its core, crystallization is a process driven by the minimization of free energy. According to the second law of thermodynamics, systems naturally evolve towards states of lower free energy, which is a measure of the energy available to do work. In the context of crystallization, this means that as a solution cools or as the concentration of solute increases, the free energy associated with the dissolved molecules becomes higher than that of the solid phase. This disparity in free energy drives the solute molecules to come together and form a crystal lattice structure, where they are more stable and have lower free energy. The Gibbs free energy equation (\(\Delta G = \Delta H - T\Delta S\)) is particularly relevant here. The change in Gibbs free energy (\(\Delta G\)) determines whether a process is spontaneous; if \(\Delta G\) is negative, the process is favorable. For crystallization to occur, \(\Delta G\) must be negative, indicating that the formation of the crystal lattice is energetically favorable compared to the dissolved state. The enthalpy term (\(\Delta H\)) represents the change in internal energy, which is often negative for crystallization due to the release of energy as bonds form in the crystal lattice. The entropy term (\(T\Delta S\)) reflects the change in disorder; since crystallization involves molecules moving from a disordered solution to an ordered lattice, entropy decreases, making \(T\Delta S\) positive. However, as temperature decreases or concentration increases, the negative \(\Delta H\) term can dominate, leading to a negative overall \(\Delta G\), thus favoring crystallization. Another critical thermodynamic concept is supersaturation. A solution becomes supersaturated when it contains more dissolved solute than it can hold at equilibrium. This state is metastable and can be achieved through cooling or evaporation. Once a nucleus forms—a process known as nucleation—the supersaturated solution rapidly precipitates out crystals as the system seeks to reduce its free energy. The rate of nucleation and subsequent crystal growth is influenced by factors such as temperature, concentration, and the presence of impurities or nucleation sites. Thermodynamic principles also explain why certain conditions are optimal for crystallization. For instance, slow cooling allows for more ordered crystal growth because it gives the system time to reach equilibrium at each temperature step, resulting in larger, more perfect crystals. Conversely, rapid cooling can lead to the formation of smaller crystals or even amorphous solids due to kinetic limitations. In summary, thermodynamic principles provide the foundational framework for understanding how and why crystallization occurs. By considering the changes in free energy, enthalpy, and entropy, scientists can predict and control the conditions necessary for crystal formation, making these principles indispensable in fields ranging from materials science to pharmaceuticals and biotechnology.
Kinetic Factors and Nucleation
Kinetic factors and nucleation are pivotal components in the process of crystallization, influencing the formation, growth, and final structure of crystals. **Kinetic factors** refer to the dynamic conditions under which crystallization occurs, including temperature, concentration of the solution, solvent properties, and agitation. These factors determine the rate at which molecules come together to form a crystal lattice. For instance, a higher concentration of solute can increase the likelihood of collisions between molecules, thereby accelerating nucleation and crystal growth. Similarly, temperature plays a crucial role; lower temperatures often slow down molecular movement, allowing for more ordered and stable crystal structures to form. The choice of solvent is also critical as it affects the solubility of the solute and can influence the shape and size of the crystals. **Nucleation**, on the other hand, is the initial step in crystallization where a small cluster of molecules (nucleus) forms around which the crystal grows. There are two types of nucleation: homogeneous and heterogeneous. **Homogeneous nucleation** occurs spontaneously within a pure solution when a critical concentration is reached, leading to the formation of a nucleus without any external influence. This process is less common due to its high energy requirements. **Heterogeneous nucleation**, more prevalent in practice, involves the presence of impurities or surfaces that act as nucleation sites, significantly lowering the energy barrier for nucleus formation. The presence of these sites can control the number and size of crystals formed, making it easier to achieve uniform crystallization. The interplay between kinetic factors and nucleation is complex and highly influential on the final product. For example, rapid cooling or high supersaturation can lead to multiple nucleation events, resulting in smaller crystals with potential defects. Conversely, slow cooling or controlled supersaturation allows for fewer but larger, more perfect crystals to form. Understanding these kinetic and nucleation processes is essential for optimizing crystallization techniques in various fields such as pharmaceuticals, materials science, and chemical engineering. By manipulating these factors, scientists can tailor crystal properties to meet specific requirements, enhancing the performance and quality of crystalline materials. In summary, kinetic factors and nucleation are fundamental to the science behind crystallization. By carefully controlling these elements, researchers can predict and influence the crystallization process to produce crystals with desired characteristics. This precision is crucial for applications ranging from drug development to advanced materials synthesis, highlighting the importance of a deep understanding of these underlying principles in achieving high-quality crystalline products.
Crystal Structure and Morphology
Crystal structure and morphology are fundamental aspects of crystallization, offering insights into the intricate arrangement and external form of crystals. At its core, the crystal structure refers to the three-dimensional arrangement of atoms, molecules, or ions within a crystal lattice. This lattice is characterized by its periodicity, meaning that the pattern of atomic positions repeats in a regular manner. The specific arrangement can be described using parameters such as lattice constants and crystal symmetry, which determine the overall properties of the crystal, including its optical, electrical, and mechanical characteristics. Morphology, on the other hand, pertains to the external shape and appearance of crystals. It is influenced by factors such as the growth conditions, including temperature, concentration of the solution, and the presence of impurities. The morphology can vary significantly even among crystals of the same substance due to these environmental factors. For instance, crystals grown in a solution with high supersaturation may exhibit more complex shapes compared to those grown under lower supersaturation conditions. Understanding both the internal structure and external morphology is crucial for predicting and controlling the properties of crystalline materials. The interplay between crystal structure and morphology is particularly evident in their impact on material properties. For example, the optical properties of a crystal can be significantly affected by its morphology; a crystal with a well-defined faceted shape may exhibit different light scattering or absorption characteristics compared to one with an irregular shape. Similarly, mechanical properties such as hardness and cleavage can be influenced by both the internal structure and external form of the crystal. In practical applications, knowledge of crystal structure and morphology is essential for optimizing crystallization processes. In pharmaceuticals, for instance, the bioavailability and efficacy of drugs can depend on the polymorphic form (different crystal structures) of the active ingredient. In electronics, the precise control over crystal morphology is critical for producing high-quality semiconductor materials. Furthermore, in geology, understanding the crystal structures and morphologies of minerals helps in identifying their origins and evolutionary histories. In conclusion, the study of crystal structure and morphology provides a comprehensive understanding of crystalline materials at both microscopic and macroscopic levels. By elucidating how atoms are arranged within a crystal lattice and how these arrangements influence the external shape, scientists can tailor crystallization processes to produce materials with desired properties. This understanding is pivotal in various fields ranging from materials science to pharmaceuticals and geology, highlighting the importance of these concepts in the broader context of crystallization science.
Applications and Techniques in Crystallization
Crystallization is a pivotal process in various industries, offering a multitude of applications and techniques that enhance product quality and efficiency. This article delves into the diverse facets of crystallization, exploring its industrial applications, laboratory techniques, and advanced technologies. On the industrial front, crystallization plays a crucial role in the production of pharmaceuticals, chemicals, and food products, ensuring purity and consistency. In laboratories, precise techniques such as solvent selection and cooling methods are employed to optimize crystallization outcomes. Furthermore, advanced technologies like continuous crystallization and sonocrystallization are revolutionizing the field by offering faster, more efficient, and scalable processes. By understanding these different aspects, we can appreciate the breadth of crystallization's impact. Let us begin by examining how crystallization is integral to industrial sectors, particularly in the production of pharmaceuticals, chemicals, and food products.
Industrial Applications: Pharmaceuticals, Chemicals, and Food
Crystallization plays a pivotal role in various industrial sectors, particularly in the production of pharmaceuticals, chemicals, and food products. In the pharmaceutical industry, crystallization is crucial for the purification and formulation of active pharmaceutical ingredients (APIs). The process ensures the production of high-purity crystals with specific physical and chemical properties, which are essential for drug efficacy and stability. For instance, the crystallization of APIs can influence their solubility, bioavailability, and shelf life. Pharmaceutical companies often employ advanced crystallization techniques such as solvent-mediated crystallization, seeding, and sonocrystallization to control crystal size, shape, and polymorphism, thereby optimizing drug performance. In the chemical industry, crystallization is used to produce a wide range of products including fertilizers, detergents, and fine chemicals. This process allows for the separation and purification of chemicals from complex mixtures, enhancing their purity and quality. For example, the crystallization of ammonium sulfate is critical in the production of fertilizers, while the crystallization of sodium chloride (common salt) is essential for various industrial applications including water treatment and manufacturing processes. Advanced crystallization techniques like continuous crystallization and cooling crystallization are often employed to improve yield and efficiency. The food industry also relies heavily on crystallization for the production of high-quality food products. Sugar refining is a classic example where crystallization is used to purify sucrose from molasses. The process involves seeding and controlled cooling to form large crystals of sugar that can be easily separated from impurities. Similarly, in the dairy industry, lactose crystallization is important for producing lactose powder used in infant formula and other dairy products. Additionally, crystallization is used in the production of food additives such as citric acid and ascorbic acid, which are essential for maintaining the flavor and nutritional value of various food items. Across these industries, the application of crystallization techniques is not only about achieving purity but also about controlling the physical properties of the final product. For instance, crystal size distribution can affect the flowability of powders in pharmaceuticals and food products, while crystal shape can influence the appearance and texture of final goods. Moreover, advancements in crystallization technology have enabled real-time monitoring and control systems that help in optimizing the crystallization process, reducing energy consumption, and improving overall product quality. In summary, crystallization is a cornerstone process in the pharmaceutical, chemical, and food industries due to its ability to purify and tailor the physical properties of materials. By leveraging advanced techniques and technologies, these industries can ensure the production of high-quality products that meet stringent regulatory standards while also optimizing efficiency and reducing costs. As research continues to advance in this field, we can expect even more sophisticated applications of crystallization that will further enhance product quality and industrial productivity.
Laboratory Techniques: Solvent Selection and Cooling Methods
In the realm of crystallization, laboratory techniques such as solvent selection and cooling methods are pivotal for achieving high-quality crystals. The choice of solvent is a critical factor because it directly influences the solubility of the compound, the rate of crystallization, and the purity of the final product. Different solvents have varying polarities and solubility parameters, which can significantly affect the solubility curve of a substance. For instance, polar solvents like ethanol or acetone are often used for compounds that are soluble in these media, while non-polar solvents such as hexane or toluene are preferred for less polar substances. Additionally, mixed solvents can be employed to tailor the solubility characteristics to the specific needs of the crystallization process. Cooling methods are another essential aspect of crystallization techniques. Cooling can be achieved through various means, each with its own advantages and limitations. Slow cooling is commonly used to promote the formation of large, well-ordered crystals by allowing sufficient time for nucleation and growth. This method involves gradually lowering the temperature of the solution over a period of time, often using a thermostatically controlled bath or a programmable cooling system. Rapid cooling, on the other hand, can lead to the formation of smaller crystals or even amorphous solids due to the rapid decrease in solubility. However, it may be necessary in certain cases where slow cooling is impractical or when specific crystal forms are desired. Evaporative cooling is another technique where the solvent is slowly evaporated under controlled conditions, allowing the solution to cool naturally as the solvent vaporizes. This method is particularly useful for volatile solvents and can be enhanced using techniques such as rotary evaporation or vacuum distillation. Furthermore, advanced cooling methods such as sonocrystallization and laser-induced crystallization have been developed to offer more precise control over the crystallization process. These techniques utilize ultrasonic waves or laser pulses to induce nucleation sites within the solution, enabling the formation of uniform crystals with specific properties. The combination of appropriate solvent selection and cooling methods is crucial for optimizing crystallization outcomes. For example, in pharmaceutical applications, the choice of solvent and cooling rate can significantly impact the polymorphic form of the drug, which in turn affects its bioavailability and stability. Similarly, in materials science, precise control over crystallization conditions is essential for producing materials with desired physical and chemical properties. In summary, solvent selection and cooling methods are fundamental laboratory techniques in crystallization that require careful consideration to achieve optimal results. By understanding the interplay between these factors and leveraging advanced technologies, researchers and practitioners can tailor their crystallization processes to produce high-quality crystals with specific characteristics, thereby enhancing the applications and efficacy of crystallized materials across various fields.
Advanced Technologies: Continuous Crystallization and Sonocrystallization
In the realm of crystallization, two advanced technologies have emerged to revolutionize the process: Continuous Crystallization and Sonocrystallization. These innovative methods not only enhance the efficiency and quality of crystalline products but also offer significant advantages over traditional batch crystallization techniques. **Continuous Crystallization** is a game-changer in the field, allowing for the continuous production of crystals without the need for batch processes. This method involves maintaining a steady state where the solution is continuously fed into a crystallizer, and the crystals are simultaneously harvested. Continuous crystallization offers several benefits, including improved control over crystal size distribution, higher purity, and reduced energy consumption. It also enables real-time monitoring and adjustment, ensuring consistent product quality. Industries such as pharmaceuticals, where precise control over crystal properties is crucial, greatly benefit from this technology. For instance, continuous crystallization can produce uniform crystals of active pharmaceutical ingredients (APIs), which is essential for ensuring the efficacy and safety of drugs. **Sonocrystallization**, on the other hand, leverages the power of ultrasound to induce nucleation and control crystal growth. By applying high-frequency sound waves to a supersaturated solution, sonocrystallization can initiate nucleation at specific points, leading to more uniform crystal formation. This technique is particularly useful in reducing the metastable zone width, thereby speeding up the crystallization process. Additionally, sonocrystallization can inhibit the formation of unwanted polymorphs and improve the overall yield of the desired crystal form. This method has found applications in various fields, including food processing and biotechnology, where it helps in producing high-quality crystals with tailored properties. For example, in the production of sugar crystals, sonocrystallization can enhance the purity and texture of the final product. Both continuous crystallization and sonocrystallization are supported by advanced analytical tools and computational models that help in optimizing the crystallization conditions. Techniques such as process analytical technology (PAT) and computational fluid dynamics (CFD) play a crucial role in monitoring and predicting the behavior of the crystallization process. These tools enable researchers and manufacturers to fine-tune parameters such as temperature, concentration, and flow rates to achieve optimal results. The integration of these advanced technologies into crystallization processes has opened new avenues for research and development. For instance, combining continuous crystallization with sonocrystallization could potentially create hybrid systems that maximize efficiency and product quality. Such innovations are driving advancements in various industries, from pharmaceuticals to materials science, by enabling the production of high-quality crystals with precise control over their properties. In summary, continuous crystallization and sonocrystallization represent significant strides in the field of crystallization. These technologies offer enhanced control, improved product quality, and increased efficiency, making them invaluable tools for industries that rely on crystalline materials. As research continues to evolve, these methods are likely to play an even more pivotal role in shaping the future of crystallization processes.