What Is Chemiosmosis
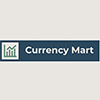
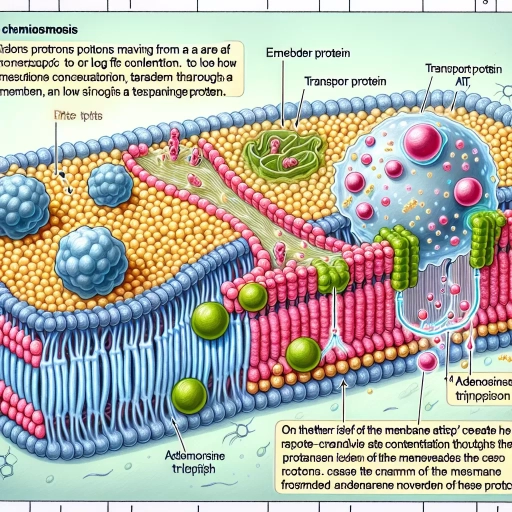
Chemiosmosis is a fundamental biological process that underpins the energy production in cells, particularly within mitochondria. This intricate mechanism involves the movement of ions across cellular membranes, generating a proton gradient that drives the synthesis of ATP, the primary energy currency of cells. To fully appreciate chemiosmosis, it is essential to delve into its core principles, understand its operational dynamics within mitochondria, and explore its broader significance and applications. In this article, we will first **Understanding the Basics of Chemiosmosis**, where we will dissect the foundational concepts that make chemiosmosis possible. We will then examine **The Process of Chemiosmosis in Mitochondria**, detailing how this process unfolds in the cellular context. Finally, we will discuss **Importance and Applications of Chemiosmosis**, highlighting its critical role in cellular metabolism and its implications for various fields of study. By grasping these aspects, readers will gain a comprehensive understanding of chemiosmosis and its pivotal role in sustaining life. Let us begin by **Understanding the Basics of Chemiosmosis**.
Understanding the Basics of Chemiosmosis
Chemiosmosis is a fundamental process in cellular biology that underpins the energy production within cells, particularly during cellular respiration. This intricate mechanism involves the movement of ions across cell membranes, generating a proton gradient that drives the synthesis of ATP, the primary energy currency of the cell. To fully grasp chemiosmosis, it is essential to delve into its definition and mechanism, understanding how it leverages chemical energy to create an electrochemical gradient. Additionally, exploring its role in cellular respiration will highlight its critical function in converting glucose into usable energy. Finally, identifying the key components involved, such as the electron transport chain and ATP synthase, will provide a comprehensive view of how chemiosmosis operates. By examining these aspects, we can gain a deeper understanding of the basics of chemiosmosis and its pivotal role in sustaining cellular life. Understanding the basics of chemiosmosis is crucial for appreciating the intricate processes that power our cells.
Definition and Mechanism
Chemiosmosis, a fundamental process in cellular respiration and photosynthesis, is defined as the movement of ions across a selectively permeable membrane, driven by the concentration gradient of those ions. This mechanism is crucial for generating ATP, the primary energy currency of cells. At its core, chemiosmosis relies on the establishment of a proton gradient across a membrane, typically the inner mitochondrial membrane in eukaryotic cells or the thylakoid membrane in chloroplasts. The process begins with the transfer of electrons through a series of electron transport chains embedded within these membranes. As electrons move through these chains, they lose energy, which is harnessed to pump protons (hydrogen ions) from one side of the membrane to the other. This creates a significant concentration gradient, with a higher concentration of protons on one side and a lower concentration on the other. The resulting electrochemical gradient has both a chemical component (the proton concentration gradient) and an electrical component (the membrane potential). The energy stored in this gradient is then utilized by the enzyme ATP synthase, which spans the membrane. ATP synthase allows protons to flow back across the membrane, down their concentration gradient, through its proton channel. This flow of protons drives the rotation of a stalk within ATP synthase, which in turn drives the synthesis of ATP from ADP and inorganic phosphate. This process is known as chemiosmotic coupling. In essence, chemiosmosis converts the energy from the electron transport chain into mechanical energy (the rotation of the stalk) and then into chemical energy (the formation of ATP). This efficient mechanism ensures that cells can produce ATP in a controlled and sustainable manner, supporting various cellular activities such as muscle contraction, transport of molecules across membranes, and biosynthesis of macromolecules. Understanding chemiosmosis is pivotal for grasping how cells generate energy from their environment and how this energy is utilized to sustain life processes.
Role in Cellular Respiration
In the intricate process of cellular respiration, chemiosmosis plays a pivotal role in harnessing energy from the food we consume. Cellular respiration is a metabolic pathway that converts glucose into ATP (adenosine triphosphate), the primary energy currency of cells. This process is divided into three main stages: glycolysis, the citric acid cycle, and oxidative phosphorylation. It is during oxidative phosphorylation that chemiosmosis comes into play. Chemiosmosis is the mechanism by which cells generate most of their ATP during cellular respiration. It occurs in the mitochondria, specifically across the inner mitochondrial membrane. Here, the electron transport chain (ETC) is embedded, consisting of a series of protein complexes that facilitate the transfer of electrons from high-energy molecules (NADH and FADH2) to oxygen. As these electrons flow through the ETC, they lose energy, which is used to pump protons (hydrogen ions) across the inner mitochondrial membrane from the mitochondrial matrix to the intermembrane space. This creates a proton gradient, or a concentration gradient, where protons accumulate in higher concentrations outside the matrix. The buildup of this proton gradient generates a significant electrochemical potential difference across the membrane. This potential energy is then harnessed by ATP synthase, an enzyme embedded in the same membrane. ATP synthase allows protons to flow back across the membrane down their concentration gradient, using this energy to drive the synthesis of ATP from ADP (adenosine diphosphate) and inorganic phosphate. This process is known as chemiosmosis because it involves the movement of ions across a selectively permeable membrane to produce chemical energy in the form of ATP. In essence, chemiosmosis is crucial for efficient energy production in cells. Without this mechanism, cells would not be able to generate sufficient ATP to meet their energy demands. The proton gradient created during chemiosmosis is so powerful that it can produce up to 32-34 ATP molecules per glucose molecule during oxidative phosphorylation, significantly more than the 2 ATP molecules produced in glycolysis and the citric acid cycle combined. This underscores the central role of chemiosmosis in cellular respiration, making it an indispensable component of cellular metabolism and energy production. Understanding chemiosmosis provides insights into how cells optimize their energy production processes, highlighting the intricate and highly efficient mechanisms that underpin life at the cellular level.
Key Components Involved
Understanding the basics of chemiosmosis hinges on grasping its key components, which are intricately linked to facilitate the generation of ATP in cells. At the heart of chemiosmosis is the **proton gradient**, a concentration gradient of protons (hydrogen ions) across a membrane, typically the inner mitochondrial membrane in eukaryotic cells or the plasma membrane in prokaryotic cells. This gradient is established through the transfer of electrons during the process of cellular respiration, specifically during the electron transport chain. Here, electrons are passed through a series of protein complexes embedded in the membrane, each complex pumping protons from one side of the membrane to the other, creating a high concentration of protons on one side. Another crucial component is **ATP synthase**, an enzyme embedded in the same membrane where the proton gradient is formed. ATP synthase harnesses the energy stored in the proton gradient to drive the synthesis of ATP from ADP and inorganic phosphate. As protons flow back across the membrane through ATP synthase, they drive the rotation of a stalk within the enzyme, which in turn drives the binding of ADP and phosphate to form ATP. This process is highly efficient, with each proton passing through ATP synthase contributing to the production of one ATP molecule. The **electron transport chain** itself is also a vital component, consisting of a series of protein complexes that facilitate electron transfer and proton pumping. These complexes include NADH dehydrogenase, cytochrome b-c1 complex, cytochrome oxidase, and others, each playing a specific role in transferring electrons and generating the proton gradient. The electrons ultimately reduce oxygen to water, completing the cycle. Additionally, **coenzymes** such as NAD+ and FAD serve as electron carriers, capturing electrons from metabolic pathways like glycolysis and the citric acid cycle and transferring them to the electron transport chain. These coenzymes are essential for initiating the electron flow that drives chemiosmosis. Lastly, the **membrane structure** itself is critical; it must be impermeable to protons to maintain the gradient. The phospholipid bilayer of the membrane, along with embedded proteins, ensures that protons can only cross through specific channels like ATP synthase, thereby conserving the energy stored in the gradient. In summary, chemiosmosis relies on a finely tuned interplay between the proton gradient, ATP synthase, the electron transport chain, coenzymes, and the membrane structure. Each component plays a unique role in harnessing energy from electron transfer reactions to produce ATP, the primary energy currency of the cell. Understanding these components is essential for appreciating how chemiosmosis underpins cellular metabolism and energy production.
The Process of Chemiosmosis in Mitochondria
Chemiosmosis, a fundamental process in cellular respiration, is the mechanism by which mitochondria generate energy for the cell. This intricate process involves the coordinated action of several key components within the mitochondrial inner membrane. At its core, chemiosmosis relies on the **Electron Transport Chain**, a series of protein complexes that facilitate the transfer of electrons from high-energy molecules to oxygen, releasing energy in the form of a proton gradient. This gradient is crucial for **Proton Gradient Formation**, where protons accumulate on one side of the membrane, creating a concentration gradient. The energy stored in this gradient is then harnessed through **ATP Synthesis**, where protons flow back across the membrane, driving the production of ATP, the primary energy currency of the cell. Understanding these interconnected steps is essential for grasping the full scope of chemiosmosis and its pivotal role in cellular metabolism. By delving into these components, we can gain a deeper appreciation for the intricate machinery that powers life at the cellular level, ultimately leading to a comprehensive understanding of the basics of chemiosmosis.
Electron Transport Chain
The Electron Transport Chain (ETC) is a pivotal component of the process of chemiosmosis in mitochondria, playing a crucial role in the generation of ATP during cellular respiration. Located in the inner mitochondrial membrane, the ETC consists of a series of protein complexes and electron carriers that facilitate the transfer of electrons from high-energy molecules to oxygen. This process is initiated when electrons from NADH and FADH2, produced during glycolysis and the citric acid cycle, are passed through a sequence of electron transport complexes (Complexes I, II, III, and IV). As these electrons move down the transport chain, they lose energy, which is harnessed to pump protons (hydrogen ions) across the inner mitochondrial membrane from the mitochondrial matrix to the intermembrane space. This proton pumping creates a significant proton gradient or chemiosmotic potential across the membrane. The establishment of this proton gradient is essential for chemiosmosis. The energy stored in this gradient is utilized by ATP synthase, another enzyme embedded in the inner mitochondrial membrane. ATP synthase allows protons to flow back across the membrane, down their concentration gradient, and in doing so, drives the synthesis of ATP from ADP and inorganic phosphate through a process known as chemiosmosis. This mechanism ensures that the energy released from electron transport is efficiently converted into a usable form for cellular processes. The efficiency and complexity of the ETC are underscored by its ability to generate a substantial amount of ATP. For every NADH molecule that donates electrons to the transport chain, approximately 2.5 ATP molecules are produced, while each FADH2 molecule results in about 1.5 ATP molecules. This high yield is critical for meeting the energy demands of cells, particularly in tissues with high metabolic rates such as muscle and brain cells. In addition to its role in energy production, the ETC also influences cellular signaling pathways and redox balance. Dysregulation or defects in components of the ETC can lead to mitochondrial dysfunction, which has been implicated in various diseases including neurodegenerative disorders and metabolic syndromes. Therefore, understanding the intricacies of the Electron Transport Chain is not only essential for comprehending chemiosmosis but also for appreciating its broader implications in cellular physiology and pathology. By harnessing the energy from electron transport to drive ATP synthesis, the ETC stands as a cornerstone of cellular energy metabolism, highlighting the intricate and efficient mechanisms that underpin life at the molecular level.
Proton Gradient Formation
Proton gradient formation is a critical component of the chemiosmosis process in mitochondria, playing a pivotal role in the generation of ATP during cellular respiration. This intricate mechanism involves the movement of protons (hydrogen ions) across the mitochondrial inner membrane, creating a concentration gradient that drives the production of ATP. Here’s how it unfolds: during the electron transport chain, electrons are passed through a series of protein complexes embedded in the mitochondrial inner membrane. As these electrons move through these complexes, they lose energy, which is harnessed to pump protons from the mitochondrial matrix into the intermembrane space. This active transport creates a significant proton gradient, with a higher concentration of protons in the intermembrane space compared to the matrix. The resulting electrochemical gradient has both a chemical component (the concentration gradient) and an electrical component (the membrane potential), collectively known as the proton motive force. The proton motive force is essential for ATP synthesis. When protons flow back across the membrane through the enzyme ATP synthase, their movement down the gradient drives the rotation of a stalk within the enzyme. This mechanical energy is converted into chemical energy as ATP synthase catalyzes the phosphorylation of ADP to ATP. This process is highly efficient, allowing cells to produce a substantial amount of ATP from the energy released during the electron transport chain. Moreover, the proton gradient also influences other mitochondrial functions. For instance, it can affect the transport of other ions and molecules across the membrane and regulate various metabolic pathways. The precise regulation of proton gradient formation is crucial for maintaining mitochondrial function and overall cellular health. In summary, proton gradient formation is a fundamental aspect of chemiosmosis in mitochondria, enabling the efficient generation of ATP through the exploitation of electrochemical gradients. This complex interplay between electron transport, proton pumping, and ATP synthesis underscores the sophisticated mechanisms that cells employ to harness energy from their environment and sustain life. Understanding these processes provides valuable insights into cellular metabolism and highlights the importance of maintaining optimal mitochondrial function for overall health and well-being.
ATP Synthesis
ATP synthesis is a critical component of the chemiosmotic process in mitochondria, where the energy generated from the electron transport chain is harnessed to produce ATP, the primary energy currency of the cell. This intricate process occurs within the mitochondria, specifically across the inner mitochondrial membrane. Here, the proton gradient established by the electron transport chain plays a pivotal role. As electrons flow through a series of protein complexes embedded in the inner membrane, protons (hydrogen ions) are pumped from the mitochondrial matrix into the intermembrane space, creating a significant proton gradient. This gradient has both a chemical and electrical component, known as the proton motive force. The enzyme ATP synthase, also located in the inner mitochondrial membrane, utilizes this proton motive force to drive the synthesis of ATP from ADP and inorganic phosphate. ATP synthase functions as a molecular motor, where the flow of protons back across the membrane through its stalk subunits causes a conformational change in its headpiece, which in turn drives the phosphorylation of ADP to ATP. The efficiency of this process is remarkable; nearly all the energy stored in the proton gradient is converted into ATP, making chemiosmosis one of the most effective energy conversion mechanisms in biology. The ATP produced during this process is then transported out of the mitochondria and distributed throughout the cell, where it fuels various cellular activities such as muscle contraction, protein synthesis, and membrane transport. In addition to its role in energy production, ATP synthesis is tightly regulated to meet the cell's energy demands. When cellular energy levels are high, ATP synthase activity can be reduced, and conversely, when energy demands increase, ATP synthase ramps up production. This dynamic regulation ensures that cells maintain optimal energy homeostasis. Understanding ATP synthesis within the context of chemiosmosis highlights the sophisticated interplay between electron transport, proton gradients, and enzymatic activity that underpins cellular metabolism. This process not only underscores the importance of mitochondria as the powerhouses of eukaryotic cells but also underscores the evolutionary adaptations that have optimized energy production in living organisms. By elucidating these mechanisms, scientists can better comprehend how cells manage energy and how dysregulation in these processes can lead to various diseases, thereby informing therapeutic strategies aimed at restoring cellular energy balance.
Importance and Applications of Chemiosmosis
Chemiosmosis, a fundamental process in cellular biology, plays a pivotal role in the production of energy within cells. This mechanism, which involves the movement of ions across cell membranes to generate ATP, is crucial for sustaining life. The importance of chemiosmosis extends beyond energy production; it significantly impacts cellular metabolism by regulating various biochemical pathways. Additionally, its applications in biotechnology and medicine are vast, contributing to advancements in fields such as bioenergy and disease treatment. In this article, we will delve into the energy production in cells facilitated by chemiosmosis, its profound impact on cellular metabolism, and its diverse biotechnological and medical applications. Understanding these aspects will provide a comprehensive insight into the significance of chemiosmosis, ultimately leading to a deeper appreciation of its underlying principles. Therefore, it is essential to begin by understanding the basics of chemiosmosis.
Energy Production in Cells
Energy production in cells is a complex and highly efficient process that underpins the survival and function of all living organisms. At the heart of this process is chemiosmosis, a mechanism that harnesses the energy from chemical reactions to generate ATP (adenosine triphosphate), the primary energy currency of the cell. In both prokaryotic and eukaryotic cells, chemiosmosis occurs across cell membranes, where it plays a crucial role in cellular respiration and photosynthesis. During cellular respiration, particularly in the mitochondria of eukaryotic cells, chemiosmosis is integral to the electron transport chain. Here, electrons are transferred through a series of protein complexes embedded in the mitochondrial inner membrane. This electron flow creates a proton gradient across the membrane, with protons accumulating in the intermembrane space. The enzyme ATP synthase then utilizes this proton gradient to drive the synthesis of ATP from ADP and inorganic phosphate. This process is not only vital for energy production but also exemplifies how chemiosmosis leverages the principles of thermodynamics to convert chemical energy into a usable form. In photosynthetic organisms such as plants and cyanobacteria, chemiosmosis occurs in the thylakoid membranes of chloroplasts. Here, light energy from sunlight is captured by pigments like chlorophyll and converted into chemical energy through the light-dependent reactions. This process generates a proton gradient across the thylakoid membrane, which is subsequently used by ATP synthase to produce ATP. Additionally, the electrons released during these reactions are used to reduce NADP+ to NADPH, which is then utilized in the Calvin cycle to synthesize glucose from CO2. The importance of chemiosmosis in energy production cannot be overstated. It is the fundamental mechanism by which cells convert environmental energy into a form that can be used to power metabolic processes. Without chemiosmosis, cells would lack the efficient means to generate ATP, leading to a significant impairment in cellular function and ultimately, the survival of the organism. Furthermore, understanding chemiosmosis has far-reaching applications in fields such as bioengineering, where it informs the design of artificial photosynthetic systems and biofuel production technologies. In medicine, insights into chemiosmosis can help elucidate the mechanisms underlying mitochondrial diseases and develop targeted therapeutic strategies. Thus, chemiosmosis stands as a cornerstone of cellular biology, highlighting the intricate and efficient ways in which cells produce energy to sustain life.
Impact on Cellular Metabolism
Chemiosmosis, the process by which cells generate energy through the movement of ions across cellular membranes, has a profound impact on cellular metabolism. This mechanism is crucial for the production of ATP (adenosine triphosphate), the primary energy currency of the cell. During chemiosmosis, protons (hydrogen ions) are pumped across the mitochondrial inner membrane or the thylakoid membrane in chloroplasts, creating a proton gradient. This gradient drives the enzyme ATP synthase to produce ATP from ADP and inorganic phosphate, a process known as oxidative phosphorylation in mitochondria and photophosphorylation in chloroplasts. The energy generated through chemiosmosis is essential for various cellular activities. It fuels the synthesis of macromolecules such as proteins, nucleic acids, and lipids, which are vital for cell growth, repair, and maintenance. Additionally, ATP is required for muscle contraction, nerve impulse transmission, and the transport of molecules across cell membranes against concentration gradients. Without chemiosmosis, cells would lack the necessary energy to perform these critical functions, leading to cellular dysfunction and potentially cell death. Moreover, chemiosmosis influences the regulation of metabolic pathways. The ATP produced during chemiosmosis can inhibit or activate key enzymes in glycolysis, the citric acid cycle, and fatty acid oxidation, thereby modulating the overall metabolic state of the cell. For instance, high ATP levels can signal the cell to reduce energy-producing pathways and increase energy-consuming processes like biosynthesis. This feedback mechanism ensures that cellular energy production is tightly regulated to meet the cell's current needs. In photosynthetic organisms, chemiosmosis plays a dual role in both light-dependent reactions and the Calvin cycle. In the light-dependent reactions, chemiosmosis generates ATP and NADPH, which are then used in the Calvin cycle to fix carbon dioxide into organic compounds. This integration of chemiosmosis with photosynthesis underscores its importance in supporting life on Earth by enabling plants and other photosynthetic organisms to convert sunlight into chemical energy. In summary, chemiosmosis is a cornerstone of cellular metabolism, providing the energy necessary for a wide range of cellular processes. Its impact extends beyond ATP production to the regulation of metabolic pathways, ensuring that cells operate efficiently and respond appropriately to their environment. The significance of chemiosmosis is evident in its role in supporting both aerobic respiration in mitochondria and photosynthesis in chloroplasts, making it a fundamental aspect of life as we know it.
Biotechnological and Medical Applications
Chemiosmosis, the process by which cells generate energy through the movement of ions across cell membranes, has profound implications for both biotechnological and medical applications. In biotechnology, chemiosmosis is crucial for the development of biofuels and bioproducts. For instance, microbial fuel cells rely on chemiosmosis to convert organic matter into electrical energy, offering a sustainable alternative to traditional fossil fuels. Additionally, understanding chemiosmosis helps in optimizing fermentation processes, which are essential for producing a wide range of products including antibiotics, vitamins, and enzymes. This optimization can lead to more efficient and cost-effective production methods. In medical applications, chemiosmosis plays a pivotal role in understanding and treating various diseases. Mitochondrial dysfunction, often linked to impaired chemiosmotic processes, is associated with neurodegenerative diseases such as Alzheimer's and Parkinson's. Research into chemiosmosis can provide insights into the molecular mechanisms underlying these conditions, potentially leading to the development of new therapeutic strategies. Furthermore, chemiosmosis is integral to the functioning of the heart and skeletal muscles; disruptions in this process can lead to conditions like muscular dystrophy and heart failure. Understanding how chemiosmosis contributes to these diseases can help in designing targeted treatments. Moreover, chemiosmosis is vital in the field of cancer research. Cancer cells often exhibit altered metabolic profiles that affect their chemiosmotic processes, making them more reliant on glycolysis for energy production even in the presence of oxygen—a phenomenon known as the Warburg effect. Targeting these altered metabolic pathways could offer novel approaches for cancer therapy. Additionally, chemiosmosis is involved in the functioning of immune cells; modulating these processes could enhance immune responses against pathogens and tumors. In gene therapy and regenerative medicine, understanding chemiosmosis can improve the efficiency of gene delivery systems. For example, certain viral vectors used in gene therapy exploit chemiosmotic gradients to facilitate the entry of genetic material into cells. Similarly, in tissue engineering, maintaining optimal chemiosmotic conditions is crucial for the survival and function of engineered tissues. Overall, the principles of chemiosmosis underpin a wide array of biotechnological and medical advancements. By continuing to elucidate the mechanisms and implications of chemiosmosis, scientists can unlock new avenues for sustainable energy production, disease treatment, and innovative therapeutic strategies, ultimately contributing to improved human health and environmental sustainability.