What Is Methylamine Used For
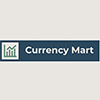
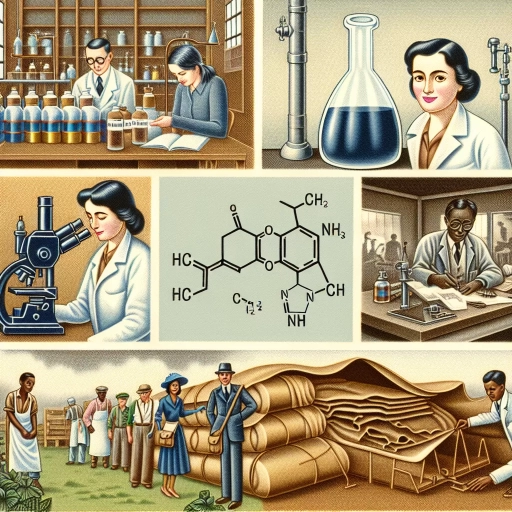
Methylamine, a versatile and potent organic compound, plays a significant role in various industrial, scientific, and environmental contexts. This article delves into the multifaceted uses of methylamine, highlighting its importance across different domains. We will explore its industrial applications, where it serves as a crucial intermediate in the production of pharmaceuticals, pesticides, and other chemicals. Additionally, we will examine its role in chemical synthesis and research, where it is used to develop new compounds and materials. Finally, we will discuss its biological and environmental uses, including its involvement in biochemical processes and its impact on ecosystems. By understanding these diverse applications, we can appreciate the breadth of methylamine's utility and its significance in modern industries. Let us begin by examining its industrial applications, where methylamine's versatility is particularly evident.
Industrial Applications of Methylamine
Methylamine, a versatile and highly reactive organic compound, plays a pivotal role in various industrial applications that span across multiple sectors. Its unique chemical properties make it an essential precursor in the manufacturing of pharmaceuticals, where it is used to synthesize a wide range of drugs and therapeutic agents. Additionally, methylamine is crucial in the production of pesticides and herbicides, enabling the creation of effective agricultural chemicals that enhance crop yields and protect against pests. Furthermore, its role in the synthesis of dyes and pigments is significant, contributing to the vibrant colors and finishes seen in textiles, paints, and other materials. Each of these applications underscores the importance of methylamine in modern industry, highlighting its multifaceted utility and indispensable nature. Transitioning to one of its most critical uses, the manufacturing of pharmaceuticals stands out as a prime example of methylamine's impact, where its reactivity is harnessed to produce life-saving medications and treatments.
Manufacturing of Pharmaceuticals
In the realm of pharmaceutical manufacturing, methylamine plays a pivotal role as a versatile intermediate in the synthesis of various drugs. The process of pharmaceutical manufacturing is intricate and highly regulated, involving multiple stages from raw material sourcing to final product formulation. Methylamine, with its unique chemical properties, is integral in the synthesis of several key pharmaceutical compounds. For instance, it is used in the production of certain antihistamines, anticholinergics, and local anesthetics. The manufacturing process typically begins with the synthesis of methylamine itself, which can be produced through the reaction of methanol with ammonia over a catalyst. Once methylamine is obtained, it can be further transformed into various intermediates through reactions such as alkylation, acylation, and amination. These intermediates are then purified and combined with other active pharmaceutical ingredients (APIs) to form the final drug product. For example, in the synthesis of certain antidepressants like phenelzine, methylamine reacts with hydrazine to form the desired compound. Similarly, in the production of antihistamines like chlorpheniramine, methylamine is used to introduce a methyl group into the molecular structure. The precision required in pharmaceutical manufacturing necessitates stringent quality control measures at every step. This includes rigorous testing for purity, potency, and stability of both the intermediates and the final product. Advanced technologies such as high-performance liquid chromatography (HPLC) and mass spectrometry are employed to ensure compliance with regulatory standards. Moreover, pharmaceutical manufacturers must adhere to Good Manufacturing Practices (GMPs) to ensure the safety and efficacy of their products. This involves maintaining sterile environments, using validated processes, and documenting all steps meticulously. The role of methylamine in this context is not just as a chemical reagent but also as a critical component that must meet stringent purity standards to avoid any adverse effects on the final drug product. In addition to its direct use in drug synthesis, methylamine also contributes indirectly by facilitating the development of new drugs through research and development. Its reactivity allows chemists to explore novel molecular structures that could lead to breakthroughs in treating various diseases. This dual role underscores the importance of methylamine in the pharmaceutical industry, making it an indispensable component in both current and future drug manufacturing processes. Overall, the integration of methylamine into pharmaceutical manufacturing highlights its versatility and critical importance in producing life-saving medications. As research continues to uncover new applications for this versatile amine, its significance in industrial processes is likely to grow, further solidifying its position as a cornerstone in the production of pharmaceuticals.
Production of Pesticides and Herbicides
The production of pesticides and herbicides is a critical industrial application of methylamine, highlighting its versatility and importance in modern agriculture. Methylamine, a primary amine derived from ammonia and methanol, serves as a key intermediate in the synthesis of various agrochemicals. For instance, it is used in the manufacture of carbamate pesticides such as carbaryl and carbofuran, which are widely employed to control insect pests in crops. These compounds work by inhibiting acetylcholinesterase, an enzyme essential for nerve function in insects, thereby providing effective pest control without significant harm to humans or the environment. In addition to pesticides, methylamine is integral in the production of herbicides like atrazine and simazine. These triazine herbicides are used extensively in corn and other cereal crops to control broadleaf weeds and grassy weeds. The synthesis involves the reaction of methylamine with cyanuric chloride, resulting in compounds that inhibit photosynthesis in plants, making them highly effective for weed management. The use of these herbicides has significantly improved crop yields by reducing competition from weeds, thereby enhancing agricultural productivity. The industrial process for producing these agrochemicals typically involves several steps. First, methylamine is synthesized through the reaction of methanol with ammonia over a catalyst. This methylamine is then reacted with other chemicals such as phosgene or cyanuric chloride to form the desired pesticide or herbicide. The resulting products are purified and formulated into various forms such as powders, granules, or liquids for easy application in agricultural settings. The role of methylamine in pesticide and herbicide production extends beyond just chemical synthesis; it also influences the environmental and health safety profiles of these products. Modern formulations often aim to minimize residues and reduce toxicity to non-target organisms while maintaining efficacy against pests. Methylamine-based agrochemicals are designed to degrade quickly in the environment, reducing long-term ecological impacts. Moreover, the use of methylamine in agrochemical production supports sustainable agriculture practices by enabling precise and targeted pest control. This reduces the need for broad-spectrum chemicals that can harm beneficial insects and other wildlife. By leveraging methylamine's reactivity and versatility, manufacturers can develop more selective and environmentally friendly pesticides and herbicides. In conclusion, the production of pesticides and herbicides using methylamine underscores its critical role in industrial applications. As a fundamental building block for these essential agrochemicals, methylamine contributes significantly to global food security by enhancing crop protection and yield. Its integration into sustainable agricultural practices further highlights its importance in balancing human needs with environmental stewardship.
Synthesis of Dyes and Pigments
The synthesis of dyes and pigments is a complex and multifaceted process that has been significantly influenced by the availability of versatile chemical intermediates like methylamine. Methylamine, with its unique properties and reactivity, plays a crucial role in the production of various dyes and pigments used across different industries. In the context of industrial applications, methylamine's ability to act as a building block or catalyst in organic synthesis makes it an indispensable component. One of the primary ways methylamine contributes to dye synthesis is through its involvement in the formation of azo dyes. Azo dyes, known for their vibrant colors and stability, are widely used in textiles, leather, and paper industries. The process typically involves the diazotization of aniline or other aromatic amines followed by coupling with a methylamine-derived compound. This reaction sequence results in the formation of azo linkages that impart the characteristic color to the dye. For instance, methylamine can be used to synthesize intermediates such as N-methyl-N-phenyl-1,4-phenylenediamine, which is then further processed to produce azo dyes like Direct Blue 15. Beyond azo dyes, methylamine is also integral in the synthesis of other types of dyes such as triarylmethane dyes and phthalocyanine pigments. Triarylmethane dyes, known for their bright colors and lightfastness, are often used in food coloring and cosmetics. Here, methylamine can participate in the alkylation reactions necessary for constructing the complex molecular structures of these dyes. Phthalocyanine pigments, renowned for their stability and intense blue-green hues, are commonly used in paints and coatings. The synthesis of these pigments involves the reaction of phthalic anhydride with urea or other nitrogen sources; methylamine can enhance this process by acting as a catalyst or by providing additional nitrogen atoms necessary for the formation of the phthalocyanine ring. In addition to its role in dye synthesis, methylamine's versatility extends to pigment production as well. Pigments such as carbon black and iron oxide are often surface-treated with methylamine to improve their dispersion properties in various media like paints, inks, and plastics. This treatment enhances the pigment's stability and color strength, making it more suitable for industrial applications. The industrial significance of methylamine in dye and pigment synthesis is further underscored by its economic viability and environmental considerations. Compared to other nitrogen-containing compounds, methylamine offers a cost-effective route to producing a wide range of dyes and pigments. Moreover, advancements in green chemistry have led to the development of more sustainable synthesis methods involving methylamine, reducing the environmental impact associated with traditional dye manufacturing processes. In conclusion, the synthesis of dyes and pigments is a critical area where methylamine's unique chemical properties make it an invaluable resource. Its role in forming azo linkages, participating in alkylation reactions, and enhancing pigment dispersion underscores its importance across various industrial sectors. As industries continue to seek more efficient and sustainable methods for producing dyes and pigments, the utility of methylamine is likely to remain paramount.
Chemical Synthesis and Research
Chemical synthesis and research are cornerstone disciplines in modern chemistry, driving innovation and advancement across various fields. At the heart of these endeavors are several key concepts that underpin the entire process. Firstly, intermediates in organic synthesis play a crucial role, as they are the transient compounds formed during the transformation of starting materials into final products. Understanding these intermediates is essential for optimizing reaction pathways and ensuring the efficiency of synthetic routes. Secondly, catalysts in chemical reactions are vital for enhancing reaction rates and selectivity, allowing chemists to achieve desired outcomes with greater precision and sustainability. Lastly, the role in polymer chemistry highlights how chemical synthesis techniques are applied to create complex macromolecules with tailored properties, which are indispensable in materials science and engineering. These interconnected aspects of chemical synthesis and research underscore the complexity and sophistication of the field. By delving into each of these areas, researchers can gain a deeper understanding of the mechanisms and methodologies that govern chemical transformations. This article will explore these themes in detail, beginning with the critical importance of **Intermediate in Organic Synthesis**.
Intermediate in Organic Synthesis
In the realm of organic synthesis, intermediates play a crucial role as they are the building blocks or transient compounds formed during the synthesis of more complex molecules. These intermediates are often pivotal in determining the efficiency, selectivity, and overall success of a synthetic pathway. In chemical synthesis and research, understanding and manipulating these intermediates is essential for several reasons. Firstly, intermediates can significantly influence the reaction mechanism and outcome. For instance, in the synthesis of pharmaceuticals, certain intermediates may be necessary to introduce specific functional groups or to achieve desired stereochemistry. The ability to control the formation and transformation of these intermediates allows chemists to tailor the synthesis process to produce the desired product with high purity and yield. Secondly, intermediates can serve as diagnostic tools to understand reaction pathways. By isolating and characterizing intermediates, researchers can gain insights into the mechanistic details of a reaction, which is invaluable for optimizing synthetic routes. This is particularly important in the development of new drugs where understanding the synthesis pathway can help in scaling up production while maintaining product quality. Thirdly, intermediates often have their own applications beyond being mere stepping stones in synthesis. For example, in organic chemistry, certain intermediates like Grignard reagents or organolithium compounds are versatile reagents that can be used in a variety of transformations. Similarly, in industrial processes, intermediates such as methylamine—a key intermediate in the production of various pharmaceuticals and agrochemicals—can be used directly or further transformed into other valuable chemicals. Moreover, the development of new intermediates can open up novel synthetic strategies. Advances in catalysis, for instance, have led to the discovery of new intermediates that facilitate more efficient and environmentally friendly syntheses. This is evident in the field of green chemistry where the use of sustainable intermediates and catalysts is becoming increasingly important to reduce waste and minimize environmental impact. In conclusion, intermediates in organic synthesis are not just transient species but are integral components that drive the field forward. Their study and manipulation are central to advancing chemical synthesis and research, enabling the development of new drugs, materials, and technologies. By understanding and leveraging these intermediates effectively, chemists can create more efficient, selective, and sustainable synthetic pathways that underpin many modern industrial processes. This underscores the importance of intermediates as a critical aspect of chemical synthesis and research.
Catalyst in Chemical Reactions
In the realm of chemical synthesis and research, catalysts play a pivotal role in facilitating and optimizing chemical reactions. A catalyst is a substance that speeds up a chemical reaction without being consumed or altered in the process. This unique property allows catalysts to be reused multiple times, making them highly efficient and cost-effective. In the context of methylamine synthesis, for instance, catalysts can significantly enhance reaction rates and yields. Catalysts work by lowering the activation energy required for a reaction to proceed, thereby increasing the rate of reaction. This can be achieved through various mechanisms such as adsorption, where reactants bind to the catalyst's surface, or by stabilizing transition states, which are intermediate stages in the reaction pathway. For example, in the synthesis of methylamine from ammonia and methanol, catalysts like zeolites or metal oxides can provide active sites that facilitate the interaction between reactants, leading to higher conversion rates and better product selectivity. The choice of catalyst is crucial and depends on the specific reaction conditions and desired outcomes. Homogeneous catalysts, which are dissolved in the reaction mixture, offer uniform distribution and high activity but can be difficult to separate from the products. Heterogeneous catalysts, on the other hand, are solid or liquid phases separate from the reactants and products, making them easier to recover and reuse. In industrial settings, heterogeneous catalysts are often preferred due to their practical advantages. Moreover, catalysts can influence the stereochemistry and regiochemistry of reactions, leading to higher purity and specificity of the final product. This is particularly important in pharmaceutical and fine chemical synthesis where precise control over molecular structure is essential. Advanced materials like nanoparticles and enzymes have also been explored as catalysts due to their high surface area and selective activity. The development of new catalysts is an active area of research, driven by the need for more efficient, sustainable, and environmentally friendly processes. Green chemistry principles emphasize the use of catalysts that are non-toxic, biodegradable, and require minimal energy input. For instance, biocatalysts derived from enzymes offer a promising avenue for sustainable synthesis routes. In summary, catalysts are indispensable tools in chemical synthesis and research, enabling faster, more efficient, and more selective reactions. Their role in optimizing methylamine production exemplifies their broader significance across various chemical processes. As research continues to advance, the development of novel catalysts will remain a key focus area for improving reaction outcomes and aligning with sustainable development goals.
Role in Polymer Chemistry
In the realm of chemical synthesis and research, polymer chemistry plays a pivotal role, particularly when it comes to understanding and manipulating the properties of polymers. Polymers, which are large molecules composed of repeating units called monomers, are ubiquitous in modern life, from plastics and fibers to adhesives and coatings. The study of polymer chemistry involves the synthesis, characterization, and application of these materials, which is crucial for advancing various technological fields. At the heart of polymer chemistry lies the synthesis process, where chemists design and create polymers with specific properties tailored for diverse applications. This involves selecting appropriate monomers, choosing the right polymerization techniques (such as addition or condensation polymerization), and controlling reaction conditions to achieve desired molecular weights and structures. For instance, in the production of polyethylene, ethylene monomers are polymerized under high pressure and temperature to form a versatile plastic used in packaging, pipes, and other consumer goods. Characterization is another critical aspect of polymer chemistry. Techniques such as nuclear magnetic resonance (NMR) spectroscopy, infrared spectroscopy (IR), and gel permeation chromatography (GPC) are employed to determine the molecular structure, composition, and size distribution of polymers. These analytical methods help researchers understand how the chemical structure of a polymer influences its physical properties, such as tensile strength, thermal stability, and solubility. The applications of polymer chemistry are vast and varied. In biomedical engineering, polymers are used to develop biocompatible materials for implants, drug delivery systems, and tissue engineering scaffolds. In the automotive industry, advanced polymers like polypropylene and polyurethane are used in vehicle components due to their durability and resistance to environmental factors. Additionally, polymers play a significant role in sustainable technologies; for example, biodegradable polymers derived from renewable resources like starch or cellulose are being developed as alternatives to traditional plastics. Furthermore, ongoing research in polymer chemistry is focused on developing smart materials that can respond to environmental stimuli such as temperature, pH, or light. These responsive polymers have potential applications in fields like drug delivery, where they can release therapeutic agents in a controlled manner based on physiological conditions. In conclusion, the role of polymer chemistry in chemical synthesis and research is multifaceted and indispensable. By understanding the principles of polymer synthesis and characterization, scientists can design materials with tailored properties that meet specific needs across various industries. As research continues to advance in this field, we can expect even more innovative applications of polymers that will shape our future technologies and improve our quality of life.
Biological and Environmental Uses
The intersection of biology and environment is a rich and dynamic field, offering numerous avenues for scientific exploration and practical application. At the heart of this discipline are three key areas that underscore its significance: biochemical studies and research, environmental remediation processes, and biodegradation and microbial metabolism. Biochemical studies and research delve into the intricate molecular mechanisms that govern biological systems, providing crucial insights into how organisms interact with their environment. Environmental remediation processes leverage these biochemical principles to develop strategies for cleaning pollutants from ecosystems, restoring balance and health to damaged environments. Meanwhile, biodegradation and microbial metabolism highlight the role of microorganisms in breaking down organic materials, a process that is both essential for ecosystem functioning and a potential tool for managing waste and pollution. By understanding these interconnected aspects, we can better appreciate the complex interplay between biological systems and their environments. This article will explore these themes in depth, beginning with a closer look at biochemical studies and research, which form the foundational knowledge necessary for advancing our understanding of biological and environmental interactions.
Biochemical Studies and Research
Biochemical studies and research play a pivotal role in understanding the multifaceted applications of methylamine, particularly in biological and environmental contexts. At the core of these studies is the elucidation of methylamine's biochemical pathways and its interactions with various biological systems. Researchers delve into the metabolic processes involving methylamine, which is a primary amine that can be derived from the breakdown of certain amino acids and other organic compounds. This understanding is crucial for identifying potential biological uses, such as in the synthesis of pharmaceuticals and agrochemicals. For instance, methylamine serves as a precursor in the production of certain drugs, including antihistamines and local anesthetics, due to its ability to form stable salts with carboxylic acids. In environmental research, biochemical studies on methylamine focus on its role in microbial metabolism and its impact on ecosystems. Methylamine is known to be a substrate for various microorganisms, which can degrade it through specific enzymatic pathways. This degradation process is significant in bioremediation efforts, where methylamine can be used to clean up contaminated sites by leveraging microbial activity. Additionally, these studies help in understanding the biogeochemical cycles involving nitrogen, as methylamine can act as a nitrogen source for certain microorganisms, influencing soil fertility and aquatic ecosystems. The biochemical properties of methylamine also make it an important tool in biochemical assays and analytical techniques. For example, methylamine can be used as a reagent in the synthesis of fluorescent probes and other biochemical markers, aiding in the detection and quantification of various biomolecules. This application is particularly valuable in molecular biology research, where precise labeling and detection are essential for understanding cellular processes. Moreover, the environmental implications of methylamine extend to its potential as a biofuel precursor. Biochemical research has shown that methylamine can be converted into methanol or other alcohols through microbial fermentation processes, offering a sustainable alternative to fossil fuels. This area of study aligns with broader efforts to develop renewable energy sources and reduce greenhouse gas emissions. In summary, biochemical studies on methylamine are fundamental to unlocking its diverse applications across biological and environmental domains. By exploring its metabolic pathways, microbial interactions, and analytical uses, researchers can harness methylamine's potential to contribute to drug development, environmental remediation, and sustainable energy solutions. These findings not only enhance our understanding of biochemical processes but also pave the way for innovative applications that benefit both human health and the environment.
Environmental Remediation Processes
Environmental remediation processes are crucial for restoring ecosystems contaminated by pollutants, and they often involve a combination of physical, chemical, and biological methods. One of the key strategies in environmental remediation is bioremediation, which leverages the metabolic capabilities of microorganisms to degrade or transform contaminants into less harmful substances. For instance, certain bacteria can break down petroleum hydrocarbons, while others can reduce heavy metals to less toxic forms. Phytoremediation, another biological approach, utilizes plants to absorb and metabolize pollutants from soil and water. This method is particularly effective for removing heavy metals and organic contaminants. Chemical remediation techniques also play a significant role. These include processes such as chemical oxidation, where oxidizing agents are introduced to break down organic pollutants, and chemical reduction, which involves the use of reducing agents to convert toxic compounds into more benign forms. Physical remediation methods, such as excavation and removal of contaminated soil or groundwater extraction followed by treatment, are often used in conjunction with biological and chemical techniques to ensure comprehensive cleanup. Advanced technologies like nanoremediation have also emerged as promising tools. Nanoparticles can be engineered to target specific contaminants, enhancing the efficiency of remediation efforts. Additionally, innovative materials such as zeolites and activated carbon are being used to adsorb pollutants from contaminated media, facilitating their removal. The selection of an appropriate remediation strategy depends on several factors, including the type and extent of contamination, site-specific conditions, and regulatory requirements. Effective environmental remediation not only mitigates immediate health risks but also helps in restoring ecological balance and preserving natural resources for future generations. In the context of methylamine uses, understanding these remediation processes is essential because methylamine itself can be a contaminant in certain industrial settings. Knowing how to remediate such contaminants is vital for maintaining environmental health and compliance with environmental regulations. In summary, environmental remediation processes are multifaceted and involve a range of biological, chemical, and physical techniques tailored to address specific types of contamination. By integrating these methods effectively, we can achieve significant improvements in environmental quality and public health. As our understanding of these processes evolves, so does our ability to protect and restore ecosystems impacted by human activities.
Biodegradation and Microbial Metabolism
Biodegradation and microbial metabolism are pivotal processes in the natural environment, playing crucial roles in the breakdown and transformation of organic compounds. Biodegradation, the decomposition of organic materials by living organisms, is primarily driven by microorganisms such as bacteria, fungi, and protozoa. These microbes utilize enzymes to break down complex molecules into simpler substances, which can then be assimilated into their metabolic pathways. This process is essential for nutrient cycling, as it releases carbon, nitrogen, phosphorus, and other elements back into the ecosystem, making them available for other organisms. Microbial metabolism is the set of biochemical reactions that occur within these microorganisms to convert substrates into energy and biomass. During biodegradation, microbes can metabolize a wide range of organic compounds, including pollutants like pesticides, industrial chemicals, and even some plastics. For instance, certain bacteria can degrade methylamine—a simple amine used in various industrial applications—by oxidizing it through specific enzymatic pathways. This metabolic activity not only helps in detoxifying the environment but also contributes to the microbial community's growth and survival. The efficiency of biodegradation is influenced by several factors, including the type of microorganism, the structure of the substrate, environmental conditions such as pH and temperature, and the availability of oxygen. Aerobic biodegradation, which occurs in the presence of oxygen, generally proceeds faster than anaerobic biodegradation. However, anaerobic conditions can still support robust microbial activity, particularly in environments like soil sediments or deep-sea vents. Biodegradation has significant biological and environmental uses. It is a key mechanism for cleaning up contaminated sites through bioremediation—a process where microorganisms are used to degrade pollutants. For example, oil spills can be mitigated by introducing oil-degrading bacteria into the affected area. Additionally, biodegradation is crucial in wastewater treatment plants where microbes break down organic waste, reducing the load on water bodies and making treated water safer for discharge. In agricultural contexts, biodegradation helps in soil health management by decomposing organic matter and recycling nutrients. This enhances soil fertility and supports plant growth without the need for synthetic fertilizers. Furthermore, understanding microbial metabolism can lead to the development of novel biotechnological applications such as biofuel production and biocatalysis. In summary, biodegradation and microbial metabolism are fundamental processes that underpin many ecological and industrial practices. By harnessing these natural processes, we can maintain ecosystem balance, mitigate pollution, and develop sustainable technologies that benefit both human society and the environment. As research continues to uncover the intricacies of microbial metabolism, its potential applications in biological and environmental contexts are likely to expand, offering promising solutions for a more sustainable future.