What Are The 5 Types Of Brain Scans
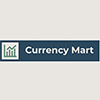
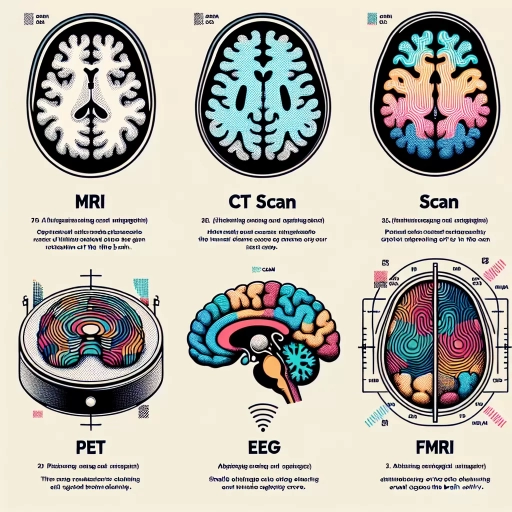
Brain scans have revolutionized the field of neuroscience, offering unparalleled insights into the human brain's structure and function. These advanced imaging techniques are crucial for diagnosing neurological disorders, understanding brain development, and guiding therapeutic interventions. In this article, we will delve into the diverse world of brain scans, exploring their significance and the various types that exist. We will begin by introducing the concept of brain scans and their importance in modern medicine, highlighting how these tools have transformed our ability to diagnose and treat brain-related conditions. Next, we will provide an overview of the five primary types of brain scans—MRI, CT, PET, EEG, and fMRI—discussing their unique characteristics and applications. Finally, we will examine advanced brain scanning techniques and future directions in this rapidly evolving field, showcasing the potential for even more precise and innovative imaging methods. By understanding these different types of brain scans and their evolving capabilities, we can better appreciate the critical role they play in advancing our knowledge of the brain and improving patient care. Let us start with an introduction to brain scans and their importance.
Introduction to Brain Scans and Their Importance
Brain scans have revolutionized the field of neuroscience, offering unparalleled insights into the human brain's structure and function. These advanced imaging techniques have evolved significantly over the years, transforming from rudimentary methods to sophisticated tools that are indispensable in modern medicine. The historical development of brain scanning techniques is a fascinating narrative that highlights the innovative spirit and scientific breakthroughs that have led to today's advanced imaging modalities. Moving beyond their historical roots, brain scans have numerous medical applications and diagnostic uses, enabling healthcare professionals to accurately diagnose and treat a wide range of neurological disorders. Furthermore, their impact on neurological research and treatment has been profound, driving new discoveries and therapeutic strategies. As we delve into the world of brain scans, it is essential to understand their historical development, which sets the stage for appreciating their current medical applications and their transformative role in neurological research and treatment. This journey begins with an exploration of the historical development of brain scanning techniques.
Historical Development of Brain Scanning Techniques
The historical development of brain scanning techniques has been a transformative journey, marked by significant advancements that have revolutionized our understanding and diagnosis of brain function and pathology. The earliest attempts to visualize the brain date back to the late 19th century with the invention of X-rays by Wilhelm Conrad Röntgen in 1895. However, these initial X-rays were limited in their ability to provide detailed images of soft tissues like the brain. A major breakthrough came with the introduction of pneumoencephalography in the early 20th century. This technique involved injecting air into the cerebral ventricles to displace cerebrospinal fluid, allowing for better contrast on X-ray images. Although it provided some insights, it was invasive and often painful. The real turning point arrived with the advent of computed tomography (CT) scans in the 1970s. Developed by Sir Godfrey Hounsfield and Allan McLeod Cormack, CT scans used computer algorithms to reconstruct detailed cross-sectional images from X-ray data. This non-invasive method significantly improved diagnostic capabilities for brain injuries, tumors, and other abnormalities. The 1980s saw the emergence of magnetic resonance imaging (MRI), pioneered by Richard Ernst and Raymond Damadian. MRI leverages magnetic fields and radio waves to generate high-resolution images of brain structures without ionizing radiation. Its superior soft-tissue contrast made it an invaluable tool for neuroimaging. Functional MRI (fMRI) further expanded our capabilities by allowing researchers to map brain activity in real-time. Developed in the 1990s, fMRI measures changes in blood flow related to neural activity, enabling scientists to study cognitive processes and neurological disorders with unprecedented precision. Positron emission tomography (PET) scans, which use radioactive tracers to visualize metabolic processes within the brain, also became integral during this period. PET scans are particularly useful for diagnosing conditions such as Alzheimer's disease and monitoring treatment efficacy. More recently, advancements in diffusion tensor imaging (DTI) have enabled detailed mapping of white matter tracts within the brain. This technique has been crucial for understanding neurological conditions like multiple sclerosis and traumatic brain injuries. Lastly, the development of functional near-infrared spectroscopy (fNIRS) offers a non-invasive method for monitoring cerebral oxygenation levels in real-time. While less detailed than other methods, fNIRS is portable and user-friendly, making it suitable for various clinical and research settings. These technological milestones have collectively transformed the field of neuroimaging from rudimentary X-rays to sophisticated tools that can map both structure and function with remarkable accuracy. As we continue to refine these techniques, we are poised to uncover even more about the intricate workings of the human brain, ultimately enhancing our ability to diagnose and treat neurological disorders effectively.
Medical Applications and Diagnostic Uses
Medical applications and diagnostic uses of brain scans are multifaceted and crucial in modern healthcare, underscoring their importance in the field of neurology and beyond. Brain scans, including MRI (Magnetic Resonance Imaging), CT (Computed Tomography) scans, PET (Positron Emission Tomography) scans, EEG (Electroencephalography), and fMRI (Functional Magnetic Resonance Imaging), serve as indispensable tools for diagnosing a wide range of neurological conditions. For instance, MRI scans are particularly effective in visualizing soft tissue structures such as the brain and spinal cord, making them ideal for detecting conditions like tumors, strokes, and multiple sclerosis. CT scans, on the other hand, are quicker and more accessible, often used in emergency settings to identify acute injuries or hemorrhages. PET scans leverage radioactive tracers to highlight metabolic activity, aiding in the diagnosis of conditions such as Alzheimer's disease and certain types of cancer. The diagnostic precision of these scans extends to various neurological disorders. EEGs, which measure electrical activity in the brain, are essential for diagnosing and monitoring epilepsy, while fMRI scans map brain function by detecting changes in blood flow, helping researchers understand cognitive processes and identify areas affected by conditions like Parkinson's disease. These technologies also play a critical role in pre-surgical planning, allowing neurosurgeons to pinpoint the exact location of tumors or other abnormalities before surgery. Furthermore, brain scans are integral in monitoring the progression of diseases and the effectiveness of treatments, enabling healthcare providers to adjust treatment plans accordingly. In addition to their diagnostic capabilities, brain scans contribute significantly to research and development in neuroscience. They help scientists understand the neural basis of behavior, cognition, and emotion, driving advancements in fields such as psychology and psychiatry. For example, fMRI studies have shed light on the neural mechanisms underlying mental health disorders like depression and anxiety, guiding the development of more targeted therapeutic interventions. The integration of brain scan data with other medical imaging techniques and genetic information is also fostering personalized medicine approaches, where treatments can be tailored to an individual's unique neurological profile. The importance of brain scans is further highlighted by their role in emergency medicine. In cases of head trauma or suspected stroke, timely and accurate imaging can be the difference between life and death. The ability to quickly identify the extent and nature of brain injuries or ischemic events allows for prompt intervention, significantly improving patient outcomes. Moreover, advancements in imaging technologies continue to enhance resolution and speed, making these diagnostic tools even more effective. In summary, the medical applications and diagnostic uses of brain scans are vast and vital. These technologies not only aid in the diagnosis and management of neurological conditions but also drive scientific research and improve patient care. As imaging techniques continue to evolve, their importance in the healthcare landscape will only continue to grow, underscoring the critical role they play in our understanding and treatment of the brain.
Impact on Neurological Research and Treatment
The advent and advancements in brain scanning technologies have profoundly impacted neurological research and treatment, revolutionizing our understanding of the brain and its functions. These tools, including MRI, CT scans, PET scans, EEG, and fMRI, have enabled researchers to visualize and study the brain in unprecedented detail. For instance, functional MRI (fMRI) allows scientists to map brain activity in real-time, providing insights into how different regions of the brain interact during various cognitive tasks. This has been instrumental in understanding neurological disorders such as Alzheimer's disease, Parkinson's disease, and stroke, enabling more accurate diagnoses and personalized treatment plans. In the realm of research, brain scans have facilitated the discovery of new biomarkers for neurological conditions. For example, PET scans can detect amyloid plaques in the brains of individuals with Alzheimer's disease, aiding in early diagnosis and monitoring disease progression. Similarly, MRI scans can identify structural changes in the brain associated with conditions like multiple sclerosis or traumatic brain injury, helping researchers to better understand disease mechanisms and develop targeted therapies. From a clinical perspective, brain scans have transformed patient care by allowing for more precise and non-invasive diagnostic procedures. CT scans are often used in emergency settings to quickly assess brain injuries or hemorrhages, while EEGs are crucial for diagnosing and managing epilepsy. The ability to visualize brain anatomy and function has also improved surgical outcomes; neurosurgeons can now use detailed images from MRI or fMRI to plan and execute complex surgeries with greater accuracy. Moreover, brain scans have opened new avenues for therapeutic interventions. For instance, transcranial magnetic stimulation (TMS), guided by fMRI, can be used to treat depression by targeting specific brain regions involved in mood regulation. Additionally, neurofeedback training, which relies on EEG feedback, helps patients manage conditions like ADHD by teaching them to control their brain activity. The integration of brain scanning technologies with other fields such as genetics and artificial intelligence further enhances their impact. For example, combining MRI data with genetic information can help identify genetic markers associated with neurological disorders, paving the way for personalized medicine approaches. AI algorithms can analyze vast amounts of imaging data to detect subtle patterns that may not be apparent to human observers, leading to more accurate diagnoses and predictive models. In summary, the impact of brain scans on neurological research and treatment is multifaceted and profound. By providing detailed insights into brain structure and function, these technologies have significantly advanced our understanding of neurological disorders, improved diagnostic accuracy, and enabled more effective therapeutic strategies. As these technologies continue to evolve, they hold great promise for further breakthroughs in neuroscience and patient care.
Types of Brain Scans: Overview and Distinctions
Brain scans are indispensable tools in modern medicine, offering a window into the intricate workings of the human brain. These advanced imaging techniques allow healthcare professionals to diagnose, treat, and understand various neurological conditions with unprecedented precision. This article delves into three primary types of brain scans: Computed Tomography (CT) scans, Magnetic Resonance Imaging (MRI), and Functional MRI (fMRI). Each of these technologies has unique principles and applications that make them invaluable in different contexts. CT scans, for instance, utilize X-rays and computer algorithms to produce detailed cross-sectional images, making them ideal for emergency situations and detecting structural abnormalities. MRI, on the other hand, leverages magnetic fields and radio waves to create high-resolution images of brain tissue, providing comprehensive insights into brain anatomy. Functional MRI takes this a step further by mapping brain activity in real-time, enabling researchers to study cognitive functions and neural pathways. By understanding the distinct capabilities of these imaging modalities, healthcare providers can tailor their diagnostic approaches to meet specific patient needs. Let's begin by exploring the principles and uses of Computed Tomography (CT) scans, a foundational tool in neuroimaging.
Computed Tomography (CT) Scans: Principles and Uses
Computed Tomography (CT) scans are a pivotal diagnostic tool in medical imaging, offering a detailed cross-sectional view of the body's internal structures. The principle behind CT scans involves the use of X-rays and computer technology to reconstruct images. Here’s how it works: a patient lies on a table that slides into a large, doughnut-shaped machine. The CT scanner emits X-rays through the body, and detectors on the opposite side measure the absorption of these X-rays by different tissues. This data is then processed by a computer to generate detailed images, often in multiple planes. CT scans are particularly useful for their versatility and speed. They can be used to diagnose a wide range of conditions, from injuries and infections to cancers and vascular diseases. In the context of brain imaging, CT scans are often the first line of investigation due to their rapid acquisition time and availability. They are especially effective in detecting acute hemorrhages, bone fractures, and certain types of strokes. For instance, a non-contrast CT scan can quickly identify bleeding in the brain, which is crucial for emergency medical decisions. Beyond emergency settings, CT scans also play a significant role in guiding interventional procedures such as biopsies and tumor treatments. Contrast agents can be administered to enhance the visibility of specific structures or abnormalities, allowing for more precise diagnoses. For example, a contrast-enhanced CT scan can help differentiate between various types of brain tumors by highlighting areas of increased blood flow. Despite the advancements in other imaging modalities like MRI, CT scans remain indispensable due to their unique strengths. They provide excellent spatial resolution and are less sensitive to patient movement compared to MRI, making them ideal for patients who may have difficulty staying still or those with certain metal implants. Additionally, CT scans are generally more accessible and cost-effective than other advanced imaging techniques. In summary, CT scans are a cornerstone of modern medical imaging, offering rapid and detailed insights into the body's internal structures. Their ability to quickly diagnose acute conditions, guide interventions, and provide high-resolution images makes them an essential tool in clinical practice, particularly in the evaluation of brain disorders. As part of the broader spectrum of brain scanning techniques, CT scans complement other modalities like MRI, PET, and SPECT by offering distinct advantages that make them invaluable in various diagnostic scenarios.
Magnetic Resonance Imaging (MRI): Detailed Imaging Capabilities
Magnetic Resonance Imaging (MRI) stands out as a premier diagnostic tool in the realm of brain scans, offering unparalleled detailed imaging capabilities. Unlike other imaging techniques such as CT scans or X-rays, MRI leverages powerful magnetic fields and radio waves to generate high-resolution images of the brain's internal structures without the use of ionizing radiation. This non-invasive method is particularly advantageous for neurological assessments, allowing clinicians to visualize both the anatomy and function of the brain with remarkable precision. One of the key strengths of MRI is its ability to differentiate between various types of soft tissues, which is crucial for diagnosing conditions such as tumors, strokes, and multiple sclerosis. The technique can produce detailed images of the brain's gray and white matter, as well as its blood vessels, making it an invaluable tool for vascular studies. Functional MRI (fMRI), a specialized variant, goes a step further by mapping brain activity in real-time, enabling researchers to study cognitive processes and identify areas of the brain involved in specific functions. MRI's versatility extends to various sequences and protocols tailored for specific diagnostic needs. For instance, T1-weighted images provide clear delineation of anatomical structures, while T2-weighted images are more sensitive to changes in tissue hydration, making them ideal for detecting edema or inflammation. Diffusion-weighted imaging (DWI) is particularly useful in acute stroke diagnosis, as it highlights areas of restricted diffusion indicative of ischemic damage. Additionally, magnetic resonance angiography (MRA) and venography (MRV) offer detailed views of blood vessels, aiding in the diagnosis of vascular malformations and other cerebrovascular conditions. The advanced capabilities of MRI also include spectroscopy, which allows for the measurement of metabolic activity within brain tissues. This can be critical in diagnosing and monitoring neurodegenerative diseases like Alzheimer's or Parkinson's, where metabolic changes precede structural alterations. Furthermore, MRI's ability to perform longitudinal studies without exposing patients to radiation makes it an ideal choice for long-term monitoring and research purposes. In summary, MRI's detailed imaging capabilities make it an indispensable tool in neuroimaging, offering a comprehensive and nuanced view of the brain's structure and function. Its non-invasive nature, high resolution, and adaptability to various diagnostic needs solidify its position as a leading modality in the arsenal of brain scanning techniques. Whether used for clinical diagnosis or research, MRI continues to advance our understanding of the brain and improve patient outcomes across a wide range of neurological conditions.
Functional MRI (fMRI): Mapping Brain Activity
Functional Magnetic Resonance Imaging (fMRI) stands out as a pivotal tool in the arsenal of neuroimaging techniques, offering unparalleled insights into the dynamic workings of the brain. Unlike structural MRI, which provides detailed images of brain anatomy, fMRI maps brain activity by detecting changes in blood flow and oxygenation levels. This method is based on the principle that active brain areas require more oxygen, leading to an increase in blood flow to those regions. The resulting signal, known as the Blood Oxygen Level-Dependent (BOLD) signal, is what fMRI captures to create functional maps of brain activity. In practice, fMRI involves placing a subject inside a powerful magnetic field and measuring the subtle changes in magnetic properties of hemoglobin as it binds to oxygen. When a brain area is active, it consumes more oxygen, which leads to a decrease in deoxyhemoglobin and an increase in oxyhemoglobin. This shift alters the magnetic properties of the blood, creating a detectable signal that is then translated into detailed maps of neural activity. These maps can reveal which brain regions are involved in various cognitive processes such as perception, attention, memory, and motor control. One of the key advantages of fMRI is its non-invasive nature, allowing researchers and clinicians to study brain function without causing harm to the subject. This has made it an indispensable tool for both basic research and clinical applications. For instance, fMRI can help diagnose neurological disorders such as stroke, epilepsy, and brain tumors by identifying areas of abnormal brain activity. It also plays a crucial role in pre-surgical planning, enabling neurosurgeons to map critical brain functions like language and motor skills before performing surgery. Moreover, fMRI has revolutionized the field of cognitive neuroscience by enabling scientists to explore complex mental processes in real-time. Studies using fMRI have provided insights into how different brain regions interact during tasks such as decision-making, problem-solving, and social cognition. This has led to a deeper understanding of neural networks and their role in various psychological and neurological conditions. Despite its many strengths, fMRI is not without limitations. The technique requires subjects to remain still during scanning to avoid motion artifacts that can distort the data. Additionally, interpreting fMRI data can be complex due to the indirect nature of the BOLD signal and the need for sophisticated statistical analysis. However, ongoing advancements in technology and methodology continue to improve the resolution and accuracy of fMRI, making it an increasingly powerful tool for understanding the intricate workings of the human brain. In summary, fMRI offers a unique window into brain function, allowing us to visualize and study neural activity with remarkable precision. Its ability to map brain activity in real-time has transformed our understanding of brain function and has significant implications for both research and clinical practice. As one of the five types of brain scans, fMRI stands out for its capacity to illuminate the dynamic interplay between different brain regions, making it an essential component in the comprehensive toolkit of neuroimaging techniques.
Advanced Brain Scanning Techniques and Future Directions
Advanced brain scanning techniques have revolutionized the field of neuroscience, offering unprecedented insights into the workings of the human brain. These cutting-edge methods not only enhance our understanding of brain function but also pave the way for future breakthroughs in diagnosis, treatment, and research. Three key techniques stand out for their innovative approaches: Positron Emission Tomography (PET) scans, which measure metabolic activity; Single Photon Emission Computed Tomography (SPECT) scans, which analyze blood flow; and Electroencephalography (EEG) and Magnetoencephalography (MEG), which monitor electrical activity. Each of these methods provides a unique window into different aspects of brain function, collectively contributing to a more comprehensive understanding of neural processes. By leveraging these technologies, scientists can delve deeper into the intricacies of brain metabolism, blood flow dynamics, and electrical signaling. This multifaceted approach is crucial for advancing our knowledge and developing new therapeutic strategies. As we explore these techniques further, we begin with Positron Emission Tomography (PET) scans, which play a pivotal role in measuring metabolic activity within the brain.
Positron Emission Tomography (PET) Scans: Metabolic Activity Measurement
Positron Emission Tomography (PET) scans represent a cutting-edge tool in the realm of advanced brain scanning techniques, offering unparalleled insights into the metabolic activity of the brain. Unlike other imaging modalities that primarily focus on structural details, PET scans leverage the principle of positron emission to map the functional and biochemical processes within the brain. This is achieved by injecting a small amount of a radioactive tracer, typically a glucose analog such as fluorodeoxyglucose (FDG), into the bloodstream. The tracer accumulates in areas of high metabolic activity, where it emits positrons that are detected by the PET scanner. This detection process allows for the creation of detailed images that highlight regions of the brain with varying levels of glucose uptake, thereby indicating areas of heightened or diminished metabolic activity. The metabolic activity measured by PET scans is particularly valuable in diagnosing and monitoring neurological conditions. For instance, in the context of neurodegenerative diseases like Alzheimer's, PET scans can reveal patterns of reduced glucose metabolism in specific brain regions, aiding in early diagnosis and tracking disease progression. Similarly, in oncology, PET scans help identify primary tumors and metastases by detecting areas with abnormally high metabolic rates, which is crucial for staging cancer and evaluating treatment efficacy. Moreover, PET scans play a pivotal role in neuroscientific research, enabling scientists to study brain function in real-time. By combining PET with other imaging techniques such as magnetic resonance imaging (MRI) or computed tomography (CT), researchers can correlate metabolic activity with structural and functional data, providing a more comprehensive understanding of brain function and behavior. This multifaceted approach has led to significant advancements in fields like neuroplasticity, cognitive neuroscience, and psychiatric disorders. Looking towards future directions, the integration of PET technology with emerging techniques such as machine learning and artificial intelligence holds immense promise. These advancements could enhance image resolution, improve diagnostic accuracy, and facilitate personalized medicine by tailoring treatments based on individual metabolic profiles. Additionally, the development of new tracers targeting specific biochemical pathways will further expand the diagnostic capabilities of PET scans, allowing for more precise monitoring of various neurological conditions. In summary, PET scans stand as a cornerstone of advanced brain scanning techniques, offering a unique window into the metabolic landscape of the brain. Their ability to measure metabolic activity makes them indispensable for both clinical diagnostics and scientific research, and their future integration with other technologies promises to revolutionize our understanding and treatment of neurological disorders. As part of the broader spectrum of brain scanning techniques, PET scans continue to drive innovation and progress in neuroscience, paving the way for groundbreaking discoveries and improved patient outcomes.
Single Photon Emission Computed Tomography (SPECT) Scans: Blood Flow Analysis
Single Photon Emission Computed Tomography (SPECT) scans are a crucial tool in the realm of advanced brain scanning techniques, particularly for analyzing blood flow and metabolic activity within the brain. This imaging modality involves the injection of a small amount of a radioactive tracer, which accumulates in areas of the brain according to blood flow and metabolic demand. The tracer emits single photons that are detected by a gamma camera, which rotates around the patient's head to capture detailed images from multiple angles. These images are then reconstructed into three-dimensional maps, providing valuable insights into regional cerebral blood flow (rCBF) and brain function. In clinical practice, SPECT scans are often used to diagnose and monitor conditions such as stroke, dementia, and epilepsy. For instance, in the case of stroke, SPECT can help identify areas of reduced blood flow, guiding therapeutic interventions aimed at restoring perfusion. In dementia, SPECT can differentiate between various types of neurodegenerative diseases by highlighting specific patterns of reduced blood flow and metabolic activity. Additionally, SPECT is useful in the evaluation of epilepsy, helping to localize seizure foci and plan surgical interventions. One of the key advantages of SPECT over other imaging modalities like PET (Positron Emission Tomography) is its accessibility and lower cost. While PET offers higher spatial resolution and sensitivity, SPECT is more widely available and can be performed with simpler equipment, making it a viable option for many clinical settings. Furthermore, SPECT tracers have longer half-lives compared to PET tracers, allowing for more flexible imaging protocols and easier integration into clinical workflows. Despite these advantages, SPECT has its limitations. The spatial resolution of SPECT images is generally lower than that of PET or MRI (Magnetic Resonance Imaging), which can make it challenging to identify small or deep-seated lesions. However, advancements in technology, such as the development of new tracers and improved detector systems, are continually enhancing the capabilities of SPECT imaging. Looking towards future directions, the integration of SPECT with other imaging modalities like MRI or CT (Computed Tomography) is becoming increasingly important. Hybrid imaging systems combine the functional information from SPECT with the anatomical detail from MRI or CT, providing a more comprehensive understanding of brain function and structure. This multimodal approach is expected to play a significant role in personalized medicine, allowing for more precise diagnosis and treatment planning. In conclusion, SPECT scans offer a powerful tool for blood flow analysis and brain function assessment, complementing other advanced brain scanning techniques. As technology continues to evolve, the role of SPECT in clinical practice and research is likely to expand, contributing to better patient outcomes and deeper insights into the complexities of brain function.
Electroencephalography (EEG) and Magnetoencephalography (MEG): Electrical Activity Monitoring
Electroencephalography (EEG) and Magnetoencephalography (MEG) are two advanced brain scanning techniques that offer unparalleled insights into the electrical activity of the brain. **EEG**, which has been in use since the early 20th century, involves the placement of electrodes on the scalp to measure the electrical activity generated by neurons. This non-invasive method is particularly useful for diagnosing and monitoring conditions such as epilepsy, sleep disorders, and encephalopathy. EEG signals can be analyzed in real-time, making it a valuable tool for clinical settings and research studies. Its high temporal resolution allows for the detection of rapid changes in brain activity, which is crucial for understanding cognitive processes like attention, perception, and decision-making. **MEG**, on the other hand, measures the magnetic fields produced by electrical currents in the brain. This technique provides a more precise localization of neural activity compared to EEG because magnetic fields are less affected by the skull and scalp. MEG is often used in conjunction with other imaging modalities like MRI to enhance spatial resolution. It is particularly beneficial for mapping brain function in relation to specific tasks or stimuli, making it an essential tool in neuroscience research. MEG's ability to detect subtle changes in neural activity makes it invaluable for studying complex brain functions such as language processing, motor control, and sensory perception. Both EEG and MEG are critical for advancing our understanding of brain function and dysfunction. They offer complementary information that can be integrated to provide a more comprehensive view of neural activity. For instance, while EEG excels at capturing rapid changes in electrical activity over time, MEG offers superior spatial resolution, allowing researchers to pinpoint the exact regions of the brain involved in various cognitive processes. This dual approach has significant implications for clinical diagnostics and therapeutic interventions, enabling more accurate diagnoses and personalized treatment plans. In terms of future directions, advancements in EEG and MEG technologies are expected to further enhance their capabilities. For example, high-density EEG systems with hundreds of electrodes are being developed to improve spatial resolution. Similarly, next-generation MEG systems with more sensitive magnetometers are on the horizon, promising even better localization of neural activity. Additionally, the integration of these techniques with other brain scanning methods like functional MRI (fMRI) and positron emission tomography (PET) will continue to refine our understanding of brain function at multiple scales. As these technologies evolve, they will play increasingly important roles in fields such as neurology, psychiatry, and cognitive neuroscience, driving innovation in both research and clinical practice. Ultimately, the synergy between EEG, MEG, and other advanced brain scanning techniques will pave the way for groundbreaking discoveries and improved patient outcomes.