What Is The Biggest Thing In The Universe
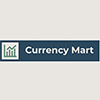
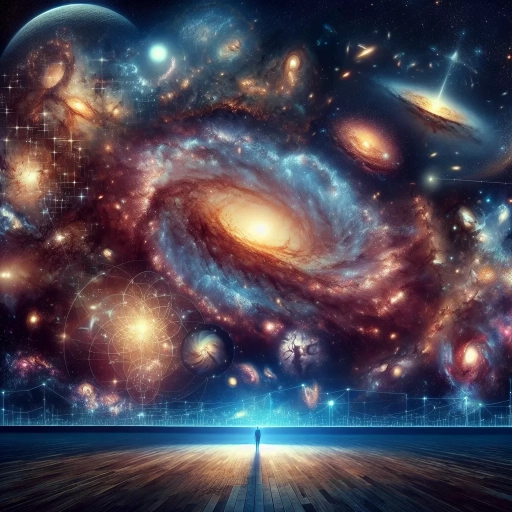
The universe, with its vast expanse and intricate complexity, has long fascinated humanity. One of the most intriguing questions in the realm of cosmology is: what is the biggest thing in the universe? This query delves into the very fabric of our understanding, requiring us to consider the scale at which we perceive the cosmos. To address this, we must first **understand the scale of the universe**, grasping the enormity of distances and sizes that define it. Next, we need to identify **candidates for the largest structures**, such as superclusters, galaxy filaments, and cosmic voids, which are contenders for this title. Finally, we must examine **theoretical and observational evidence** that supports or refutes these candidates, leveraging both scientific theories and empirical data to reach a conclusive answer. By exploring these aspects, we can gain a deeper insight into what truly constitutes the largest entity within our universe. Let us begin by **understanding the scale of the universe**, a foundational step in our journey to uncover the biggest thing in existence.
Understanding the Scale of the Universe
Understanding the scale of the universe is a profound and complex endeavor that has captivated human imagination for centuries. To grasp this vast expanse, we must delve into several key areas. First, **Defining Large-Scale Structures** helps us comprehend the intricate web of galaxy clusters, superclusters, and voids that form the cosmic landscape. This understanding is crucial for mapping the universe's overall architecture. Next, **Comparing Galactic and Cosmic Scales** allows us to appreciate the relative sizes and distances between galaxies, galaxy clusters, and other celestial entities, providing a deeper insight into the hierarchical structure of the cosmos. Finally, **The Role of Observational Astronomy** highlights the essential tools and techniques astronomers use to measure and study these vast scales, from telescopes that peer into distant galaxies to sophisticated instruments that detect subtle cosmic signals. By exploring these facets, we can gain a comprehensive understanding of the universe's scale, revealing the awe-inspiring dimensions and intricate details that define our cosmic home. This article will guide you through these critical aspects, ultimately leading to a profound appreciation of **Understanding the Scale of the Universe**.
Defining Large-Scale Structures
Defining large-scale structures in the universe involves understanding the intricate web of galaxy clusters, superclusters, and cosmic voids that span vast distances across space. These structures are not random; they are part of a larger network known as the cosmic web, which is shaped by gravitational forces and dark matter. Galaxy clusters, for instance, are the largest known gravitationally bound structures in the universe, consisting of hundreds to thousands of galaxies held together by gravity. These clusters are often found at the intersections of filaments—long, thread-like structures composed of galaxies and galaxy clusters that crisscross the universe. Superclusters take this organization to an even larger scale, comprising multiple galaxy clusters and superclusters that can stretch over a billion light-years. The most famous example is the Laniakea Supercluster, which contains our own Milky Way galaxy and extends over 500 million light-years across. These superclusters are separated by vast cosmic voids, regions of space where there are few or no galaxies. These voids can be as large as 1 billion light-years in diameter and play a crucial role in understanding the overall structure of the universe. The formation and distribution of these large-scale structures are influenced by dark matter, an invisible form of matter that does not emit, absorb, or reflect any electromagnetic radiation but has a profound impact on gravitational dynamics. Dark matter acts as a scaffold for normal matter to clump onto, facilitating the formation of galaxies and galaxy clusters. The study of large-scale structures also provides insights into the early universe and its evolution over billions of years. By analyzing the distribution and properties of these structures, scientists can infer details about the universe's age, composition, and fundamental physical laws. Understanding large-scale structures is essential for grasping the scale of the universe. It helps us visualize how galaxies are not isolated entities but are part of a much larger cosmic landscape. This perspective underscores the interconnectedness of all matter in the universe and highlights the dynamic interplay between gravity, dark matter, and normal matter that shapes our cosmos. By exploring these structures, we gain a deeper appreciation for the complexity and beauty of the universe on its grandest scales.
Comparing Galactic and Cosmic Scales
When delving into the vast expanse of the universe, it is crucial to distinguish between galactic and cosmic scales, as each offers a unique perspective on the grand tapestry of existence. At the galactic scale, we are dealing with individual galaxies—massive, gravitationally bound systems consisting of stars, stellar remnants, interstellar gas, dust, and dark matter. Our own Milky Way galaxy, for instance, is estimated to contain between 200 and 400 billion stars, each with its own planetary system. Galaxies come in various shapes and sizes, from spiral galaxies like the Milky Way to elliptical and irregular galaxies. Understanding the structure and evolution of galaxies provides insights into star formation, black hole activity, and the distribution of matter within these cosmic islands. On a much larger cosmic scale, we enter the realm of galaxy clusters and superclusters. Galaxy clusters are the largest known structures in the universe, comprising hundreds to thousands of galaxies held together by gravity. These clusters are not isolated entities but are part of even larger networks known as superclusters, which stretch across vast distances. The cosmic web, a term coined to describe this interconnected network of galaxy filaments and voids, illustrates how galaxies are distributed in a web-like pattern across the universe. This scale reveals the universe's large-scale structure and the role of dark matter and dark energy in shaping it. The cosmic scale also encompasses phenomena such as cosmic microwave background radiation, which is the residual heat from the Big Bang, and the expansion of the universe itself. Observations of this expansion have led to our current understanding of the accelerating universe, driven by dark energy. Additionally, cosmic scales involve the study of distant quasars and gamma-ray bursts, which provide windows into the early universe and extreme astrophysical processes. Comparing these scales highlights the hierarchical nature of the universe's structure. From individual stars within galaxies to galaxy clusters and superclusters, each level of organization offers a different lens through which we can understand the universe's complexity and evolution. By bridging these scales—from the local to the cosmic—we gain a more comprehensive understanding of how different components interact and influence one another, ultimately revealing the intricate beauty and vastness of the cosmos. This multi-scale approach is essential for a holistic understanding of what constitutes the biggest thing in the universe—a question that continues to inspire scientific inquiry and awe-inspiring discoveries.
The Role of Observational Astronomy
Observational astronomy plays a pivotal role in our understanding of the scale of the universe, serving as the foundational pillar upon which all astronomical knowledge is built. By meticulously observing celestial objects and phenomena, astronomers gather crucial data that helps in mapping the vast expanse of space and time. This discipline involves the systematic study of the night sky, employing a range of sophisticated instruments from ground-based telescopes to space-based observatories. These tools allow scientists to capture detailed images, spectra, and other forms of data from distant stars, galaxies, and even the cosmic microwave background radiation—the residual heat from the Big Bang. Observational astronomy is not merely about collecting data; it is an intricate process that involves careful planning, precise measurements, and rigorous analysis. Astronomers use various techniques such as photometry to measure the brightness of stars, spectroscopy to analyze their chemical composition, and interferometry to achieve high-resolution images of distant objects. These methods enable researchers to determine distances, ages, and properties of celestial bodies, thereby constructing a comprehensive picture of the universe's structure and evolution. One of the most significant contributions of observational astronomy is its ability to challenge and refine theoretical models. For instance, observations of supernovae led to the discovery of dark energy—a mysterious force driving the accelerating expansion of the universe. Similarly, detailed observations of galaxy rotations provided evidence for dark matter, an invisible form of matter that influences gravitational dynamics but does not emit light. Moreover, observational astronomy has been instrumental in expanding our understanding of cosmic scales. By observing the redshift of light from distant galaxies, astronomers have confirmed the expanding nature of the universe. The Hubble Space Telescope's observations of Cepheid variable stars in nearby galaxies helped establish a reliable distance ladder, allowing scientists to measure distances across vast intergalactic distances. These findings have collectively contributed to our current understanding that the observable universe spans approximately 93 billion light-years in diameter. In conclusion, observational astronomy is indispensable for grasping the scale of the universe. Through meticulous observations and advanced technological capabilities, astronomers continue to uncover new insights into the cosmos, refining our understanding of its vastness and complexity. As new missions like the James Webb Space Telescope come online, they promise to reveal even more about the universe's earliest moments and its ongoing evolution, further illuminating the grand tapestry that is our cosmos.
Candidates for the Largest Structures
In the vast expanse of the universe, there exist structures so enormous that they challenge our understanding of space and time. These gargantuan formations are not just mere aggregations of matter; they are the very fabric that shapes our cosmic landscape. This article delves into the largest structures known to science, exploring their nature, significance, and the insights they provide into the universe's grand design. We will examine **Superclusters and Galaxy Filaments**, the intricate networks that connect galaxies across vast distances, highlighting their role in the universe's large-scale structure. Next, we will discuss **The Sloan Great Wall**, one of the most massive known structures in the universe, which stretches over a billion light-years and offers a glimpse into the universe's early formation. Finally, we will explore **Cosmic Voids and Their Significance**, the vast empty regions that punctuate the universe, revealing how these voids contribute to our understanding of cosmic evolution. By understanding these colossal structures, we gain a deeper appreciation for the scale and complexity of the universe, ultimately leading us to a profound **Understanding of the Scale of the Universe**.
Superclusters and Galaxy Filaments
Superclusters and galaxy filaments represent the largest known structures in the universe, forming an intricate web-like network that spans billions of light-years. These colossal formations are part of the cosmic web, a term coined to describe the vast, interconnected system of galaxy clusters, superclusters, and filaments that crisscross the universe. Galaxy filaments are long, thread-like structures composed of numerous galaxies and galaxy clusters, held together by gravity and dark matter. These filaments can stretch for millions of light-years, often connecting superclusters—large groups of galaxy clusters that are gravitationally bound together. The existence of these structures was first proposed by cosmologists in the 1980s as a way to explain the observed distribution of galaxies on large scales. Since then, extensive observations and simulations have confirmed their presence. For instance, the Sloan Great Wall, discovered in 2003, is one such supercluster that spans over 1.37 billion light-years across, making it one of the largest known structures in the universe. Similarly, galaxy filaments like the Hercules-Corona Borealis Great Wall are vast networks that weave through space, providing a framework for understanding how matter is distributed on cosmic scales. These megastructures are not static; they are dynamic and evolving due to gravitational interactions and the expansion of the universe. The formation of superclusters and filaments is closely tied to the distribution of dark matter, which provides the gravitational scaffolding necessary for normal matter to clump together into galaxies and larger structures. Observational evidence from surveys like the Sloan Digital Sky Survey (SDSS) and simulations such as the IllustrisTNG project have provided detailed insights into how these structures form and evolve over billions of years. Understanding superclusters and galaxy filaments is crucial for cosmology because they offer a window into the early universe's conditions and the fundamental laws governing its evolution. By studying these structures, scientists can gain insights into dark matter's role, the distribution of normal matter, and how gravitational forces shape the cosmos on its largest scales. Moreover, these studies help refine models of cosmic evolution, ensuring a more accurate understanding of how our universe came to be as we observe it today. In summary, superclusters and galaxy filaments are among the most impressive and complex structures in the universe, reflecting both its vast scale and intricate organization. As candidates for the largest structures known, they underscore our ongoing quest to comprehend the universe's grand architecture and its underlying physical principles.
The Sloan Great Wall
The Sloan Great Wall, discovered in 2003, is a monumental structure that stands as one of the largest known entities in the universe. Spanning an astonishing 1.37 billion light-years across, this vast wall of galaxies dwarfs many other cosmic structures, making it a prime candidate for the title of the biggest thing in the universe. Named after the Sloan Digital Sky Survey (SDSS) that first identified it, this gargantuan wall is composed of countless galaxies, galaxy clusters, and superclusters, all interconnected in a web-like pattern. The sheer scale of the Sloan Great Wall is awe-inspiring; to put it into perspective, it stretches over 1/8th of the observable universe's diameter. The formation of such an enormous structure is deeply rooted in the early universe's gravitational dynamics. During the Big Bang, tiny fluctuations in density led to the eventual clumping of matter into larger and larger structures. Over billions of years, these clumps coalesced into galaxies and galaxy clusters, which then aggregated into superclusters. The Sloan Great Wall represents a culmination of this process on a cosmic scale, with its galaxies and galaxy clusters aligned in a vast, two-dimensional sheet. Despite its enormity, the Sloan Great Wall is not a solid, continuous structure but rather a network of interconnected galaxy filaments separated by vast voids. These voids are regions of space where the density of galaxies is significantly lower than average, creating an intricate pattern that underscores the dynamic nature of cosmic evolution. The study of such megastructures provides invaluable insights into the universe's large-scale structure and the distribution of matter on a universal scale. In comparison to other contenders for the largest structures in the universe, such as superclusters like Laniakea or the Hercules-Corona Borealis Great Wall, the Sloan Great Wall holds its own due to its immense size and well-defined boundaries. Its discovery has significantly expanded our understanding of cosmic architecture and has sparked further research into how these megastructures form and evolve over time. In conclusion, the Sloan Great Wall is an extraordinary example of the universe's grand design, showcasing the intricate web of galaxy distributions that underpin our cosmos. As scientists continue to explore and map these vast structures, they uncover more about the fundamental laws governing the universe's evolution and the mysteries that lie beyond our current understanding. The Sloan Great Wall stands as a testament to the awe-inspiring scale and complexity of the universe, making it an essential component in discussions about what constitutes the biggest thing in existence.
Cosmic Voids and Their Significance
Cosmic voids are vast, nearly empty regions of space that punctuate the universe, playing a crucial role in our understanding of cosmic structure and evolution. These voids are not completely devoid of matter but contain significantly fewer galaxies and other celestial objects compared to the average density of the universe. They can span millions of light-years across, making them among the largest known structures in the cosmos. The significance of cosmic voids lies in their ability to provide insights into the early universe and the distribution of matter on a grand scale. One key aspect of cosmic voids is their role in the web-like structure of the universe, often referred to as the cosmic web. This web consists of galaxy filaments and superclusters that crisscross the universe, with voids acting as the "empty" spaces between these dense regions. By studying these voids, scientists can gain a better understanding of how matter clumped together under gravity to form galaxies and galaxy clusters. The distribution and properties of voids also offer clues about the nature of dark matter and dark energy, which are essential components in models of the universe's evolution. Moreover, cosmic voids serve as important tools for cosmological research. They can be used to test models of gravity and the expansion of the universe. For instance, the distribution of voids can help scientists distinguish between different theories of gravity, such as general relativity and alternative theories like MOND (Modified Newtonian Dynamics). Additionally, the shapes and sizes of voids can provide evidence for or against various models of dark energy, which drives the accelerating expansion of the universe. The study of cosmic voids has also led to interesting observations about galaxy formation and evolution. Galaxies within voids often exhibit different properties compared to those in denser regions. For example, galaxies in voids tend to be smaller and less massive, suggesting that the environment plays a significant role in shaping galaxy evolution. This contrasts with galaxies in denser regions, which are more likely to be large and massive due to frequent mergers and interactions. In summary, cosmic voids are not just empty spaces but are integral components of the cosmic landscape. Their study offers profound insights into the structure, evolution, and fundamental physics of the universe. As candidates for the largest structures in the universe, cosmic voids underscore the complexity and scale of cosmic architecture, highlighting the intricate web of matter and energy that defines our cosmos.
Theoretical and Observational Evidence
The study of the universe's origins and evolution is deeply rooted in both theoretical and observational evidence, which collectively provide a comprehensive understanding of cosmic phenomena. This article delves into three pivotal areas that have significantly advanced our knowledge: redshift and distance measurements, cosmic microwave background data, and simulations with predictive models. By examining redshift and distance measurements, scientists have been able to map the vast expanse of the universe, tracing the expansion of galaxies and stars. The cosmic microwave background data offers a snapshot of the universe in its earliest moments, revealing crucial details about its composition and temperature. Additionally, advanced simulations and predictive models allow researchers to forecast future cosmic events and validate existing theories. These lines of evidence not only corroborate each other but also form a robust framework for understanding the scale of the universe, enabling us to grasp the enormity and complexity of the cosmos with greater precision. Understanding the scale of the universe is a fundamental quest that continues to drive scientific inquiry and inspire new discoveries.
Redshift and Distance Measurements
In the realm of cosmology, understanding the vast expanse of the universe hinges on precise measurements of redshift and distance. Redshift, a fundamental concept in astronomy, refers to the phenomenon where light from distant galaxies appears shifted towards the red end of the spectrum due to the expansion of space itself. This observation is a cornerstone of modern cosmology, providing strong evidence for the Big Bang theory. The farther away a galaxy is, the faster it is moving away from us, and thus, the more its light is shifted towards the red end of the spectrum. By measuring this redshift, astronomers can infer how fast these galaxies are receding and, consequently, how far away they are. Distance measurements in cosmology are complex and multi-layered. The closest galaxies can be measured using parallax methods, where the apparent shift of nearby stars against more distant stars is observed over the course of a year. However, for more distant galaxies, other methods must be employed. One such method involves using Cepheid variable stars, whose brightness varies predictably with their period of variation. By calibrating these stars in nearby galaxies and then observing them in more distant ones, astronomers can estimate distances with reasonable accuracy. For even more distant objects, such as supernovae or active galactic nuclei (AGN), different techniques are necessary. Type Ia supernovae are particularly useful because they all have similar maximum brightnesses, making them "standard candles" that allow astronomers to calculate their distances based on how bright they appear from Earth. Similarly, the Tully-Fisher relation and the Fundamental Plane relation provide ways to estimate distances based on the properties of galaxies themselves. The integration of these methods forms a cosmic distance ladder, each rung building upon the previous one to extend our reach into the vast expanse of space. Redshift measurements complement these distance estimates by providing a velocity component that, when combined with Hubble's Law (which relates velocity to distance), allows for the calculation of distances on a universal scale. Observational evidence supporting these measurements comes from various surveys and missions. For instance, the Hubble Space Telescope has played a pivotal role in refining our understanding of cosmic distances through its observations of Cepheid variables and supernovae. More recently, missions like the Sloan Digital Sky Survey (SDSS) and the Dark Energy Survey (DES) have mapped large portions of the sky, providing extensive data on galaxy distributions and redshifts that further solidify our understanding of cosmic distances. In summary, the interplay between redshift and distance measurements forms the backbone of modern cosmological research. By leveraging a combination of observational techniques and theoretical frameworks, scientists continue to refine our understanding of the universe's scale and evolution, ultimately contributing to our quest to comprehend what is indeed the biggest thing in the universe.
Cosmic Microwave Background Data
The Cosmic Microwave Background (CMB) data stands as a cornerstone of modern cosmology, providing irrefutable evidence for the Big Bang theory and offering insights into the universe's earliest moments. Discovered in 1964 by Arno Penzias and Robert Wilson, the CMB is the residual heat from the Big Bang, detectable in the form of microwave radiation that permeates the universe. This faint glow, averaging about 2.725 degrees Kelvin, is a snapshot of the universe when it was just 380,000 years old, a time known as the "recombination era" when electrons and protons first combined to form neutral atoms. The precision with which CMB data has been measured, particularly by satellites like COBE, WMAP, and Planck, has revolutionized our understanding of cosmic evolution. These observations have mapped the tiny fluctuations in temperature and polarization across the sky, revealing the seeds of galaxy formation and the distribution of matter and energy in the universe. The CMB's blackbody spectrum, confirmed to high accuracy, is a direct prediction of the Big Bang model. Moreover, the minute variations in temperature—on the order of one part in 100,000—correspond to the density fluctuations that eventually led to the formation of galaxies and galaxy clusters. The CMB data also provides strong evidence for the inflationary model of the universe, which posits that the universe underwent a rapid expansion in its very early stages. The observed homogeneity and isotropy of the CMB on large scales, along with the specific patterns of fluctuations, are consistent with predictions from inflationary theories. Additionally, the CMB's polarization patterns offer a window into the universe's magnetic fields and gravitational waves, further enriching our understanding of cosmic dynamics. In terms of observational evidence, the CMB data aligns seamlessly with other cosmological observations, such as the abundance of light elements and the large-scale structure of the universe. This multi-faceted approach has solidified the Big Bang theory as the most accurate description of the universe's origins. The CMB serves as a cosmic fossil record, encapsulating the conditions and processes that shaped our universe from its inception. As ongoing and future missions continue to refine these measurements, the CMB remains an indispensable tool for cosmologists, offering unparalleled insights into the universe's evolution and its ultimate fate.
Simulations and Predictive Models
Simulations and predictive models play a crucial role in understanding the vast expanse of the universe, particularly when exploring the question of what constitutes the biggest thing within it. These tools are essential for bridging the gap between theoretical frameworks and observational evidence, allowing scientists to make informed predictions and validate hypotheses. In cosmology, simulations such as the IllustrisTNG project and the EAGLE simulations recreate the evolution of the universe from the Big Bang to the present day, incorporating complex processes like galaxy formation, star birth, and black hole growth. These simulations help researchers predict how large-scale structures like galaxy clusters and superclusters form and evolve over billions of years. By comparing simulation outputs with observational data from telescopes and space missions, scientists can refine their understanding of cosmic phenomena and identify potential discrepancies that may indicate new physics or unaccounted-for factors. Predictive models, on the other hand, are used to forecast future observations and test hypotheses against empirical evidence. For instance, models predicting the distribution of dark matter and dark energy in the universe can be tested against data from gravitational lensing observations or cosmic microwave background radiation studies. These models also help in understanding the dynamics of supermassive black holes at the centers of galaxies and how they influence their surroundings. The synergy between simulations and predictive models is particularly evident in the study of cosmic voids—the vast regions of empty space that separate galaxy clusters. Simulations predict the size and distribution of these voids, which can then be verified by observational surveys like the Sloan Digital Sky Survey (SDSS). This interplay not only confirms our current understanding but also highlights areas where further research is needed. Moreover, advanced computational techniques such as machine learning algorithms are being integrated into these simulations to improve their accuracy and efficiency. Machine learning can help in identifying patterns in large datasets that might be missed by traditional methods, thereby enhancing our predictive capabilities. In summary, simulations and predictive models are indispensable tools for cosmologists seeking to understand the biggest structures in the universe. By generating detailed forecasts that can be compared with observational evidence, these tools enable scientists to refine their theories and gain deeper insights into the nature of the cosmos. This iterative process of simulation, prediction, and observation forms the backbone of modern cosmological research, driving our understanding forward with each new discovery.