What Does A Star Look Like Up Close
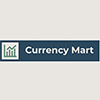
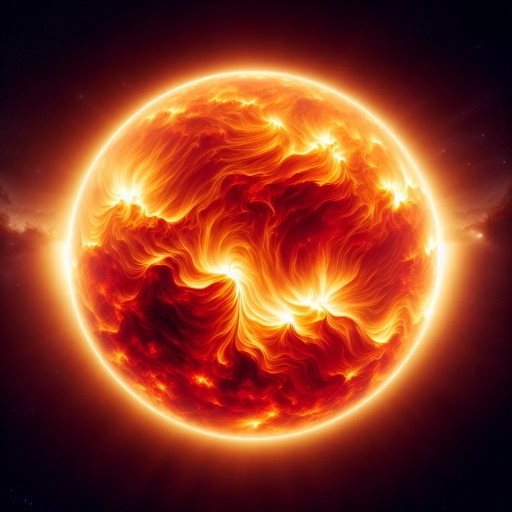
When we gaze up at the night sky, stars appear as tiny, twinkling dots, but what do they look like up close? This question has fascinated astronomers and scientists for centuries. To answer it, we must delve into several key areas. First, we need to understand the **Observational Challenges and Theoretical Models** that scientists face when trying to study stars in detail. These challenges include the immense distances between Earth and other stars, as well as the limitations of current observational technology. Next, we will explore the **Physical Characteristics of Stars**, which include their size, temperature, and composition, all of which play a crucial role in determining their appearance. Finally, we will examine **Up-Close Observations: What We Know So Far**, where we discuss the latest findings from advanced telescopes and space missions that have managed to capture detailed images of stars. By combining these perspectives, we can gain a comprehensive understanding of what a star looks like up close. Let's begin by addressing the **Observational Challenges and Theoretical Models** that form the foundation of our inquiry.
Observational Challenges and Theoretical Models
Observational challenges and theoretical models are at the heart of modern astrophysics, driving our understanding of the universe. However, these endeavors are not without their hurdles. One of the primary obstacles is the **Limitations of Current Telescopic Technology**, which restricts our ability to observe distant or faint celestial objects. Additionally, **The Role of Computational Simulations** has become increasingly crucial in bridging the gap between observations and theoretical predictions, allowing scientists to model complex phenomena that are difficult to study directly. Historically, **Historical Theories and Predictions** have laid the groundwork for current research, providing a framework for interpreting new data and refining our understanding of the cosmos. As we delve into these topics, it becomes clear that addressing the limitations of our current telescopic technology is a critical first step in advancing our knowledge of the universe.
Limitations of Current Telescopic Technology
Despite significant advancements in telescopic technology, several limitations hinder our ability to observe stars up close. One major constraint is the diffraction limit, which dictates that the resolution of a telescope is inversely proportional to its aperture size. Even with the largest ground-based telescopes, such as the Extremely Large Telescope (ELT), the diffraction limit imposes a fundamental barrier to resolving fine details on distant stars. Atmospheric interference further complicates ground-based observations, as Earth's atmosphere distorts and scatters light, reducing image quality. Space-based telescopes like the Hubble Space Telescope avoid this issue but are limited by their own design constraints and the challenges of maintaining and upgrading them. Another significant limitation is the light-gathering capability of telescopes. The faintness of distant stars means that even the most powerful telescopes struggle to collect enough light to form detailed images. This is particularly problematic for observing red dwarfs or other low-luminosity stars, which emit very little light compared to brighter counterparts like blue giants. Additionally, the dynamic range of current detectors often falls short of capturing both the bright and faint regions of a star's surface simultaneously, leading to either overexposure or underexposure. Technological limitations also extend to spectroscopic observations. While spectroscopy allows us to analyze the chemical composition and physical properties of stars, current instruments may not have the necessary resolution or sensitivity to detect subtle variations in spectral lines that could reveal detailed information about stellar atmospheres and internal dynamics. Furthermore, observational challenges are compounded by theoretical models that rely on assumptions about stellar structure and evolution. These models, while sophisticated, are based on simplifications and may not fully capture the complexities of real stellar environments. For instance, convective zones within stars can exhibit chaotic behavior that is difficult to model accurately, leading to uncertainties in predicting surface features and activity levels. Lastly, the sheer distance between Earth and other stars poses a significant challenge. Even at high speeds like those achieved by spacecraft, it would take thousands of years to reach even the nearest star system, Proxima Centauri. This distance makes direct imaging or in-situ exploration of stars impossible with current technology, forcing astronomers to rely on indirect methods that are inherently less precise. In summary, while modern telescopes have revolutionized our understanding of stars, they are constrained by physical limits such as diffraction, atmospheric interference, light-gathering capabilities, detector technology, and theoretical modeling uncertainties. Overcoming these limitations will require continued innovation in telescope design, detector technology, and computational modeling to provide a clearer picture of what stars look like up close.
The Role of Computational Simulations
In the pursuit of understanding what a star looks like up close, computational simulations play a pivotal role in bridging the gap between observational challenges and theoretical models. These simulations are sophisticated computational tools that mimic the physical processes occurring within stars, allowing scientists to predict and analyze phenomena that are difficult or impossible to observe directly. By leveraging advanced algorithms and high-performance computing, researchers can simulate the intricate dynamics of stellar interiors, including nuclear reactions, convective flows, and magnetic field interactions. These simulations help in validating theoretical models by comparing their outputs with observational data, thereby refining our understanding of stellar structure and evolution. For instance, computational simulations can recreate the conditions at the core of a star, where temperatures and pressures are so extreme that they cannot be replicated in laboratory settings. This enables scientists to study the synthesis of elements through nuclear reactions and the transport of energy through radiative and convective processes. Additionally, simulations can predict the behavior of stars under various conditions, such as during supernovae explosions or when they are part of binary systems, providing insights into complex astrophysical phenomena. By integrating observational data with simulation results, researchers can construct more accurate models of stellar life cycles, from birth to death, and gain a deeper understanding of the universe's most luminous objects. Thus, computational simulations are indispensable in astrophysics, serving as a powerful bridge between theory and observation, and significantly enhancing our ability to visualize and comprehend the intricate details of stars up close.
Historical Theories and Predictions
Historical theories and predictions have significantly shaped our understanding of what a star looks like up close, despite the observational challenges. Early astronomers like Aristarchus of Samos proposed heliocentric models, suggesting that stars are distant suns, but it wasn't until the 19th century that spectroscopy allowed scientists to analyze the light from stars. This led to the development of stellar classification systems, such as the Harvard Spectral Classification Scheme, which categorizes stars based on their surface temperatures. Theoretical models like the Lane-Emden equation and the Vogt-Russell theorem provided frameworks for understanding stellar structure and evolution. However, these models faced challenges in predicting the internal dynamics and surface features of stars. The introduction of quantum mechanics and nuclear physics in the 20th century revolutionized stellar theory, enabling predictions about stellar lifecycles, including main sequence, red giant, and white dwarf phases. Despite these advancements, direct observation of a star's surface remains elusive due to their immense distances and the limitations of current telescopic technology. Thus, theoretical models continue to play a crucial role in bridging the gap between what we can observe and what we predict about the appearance and behavior of stars up close. These predictions are continually refined by new data from space missions like the Hubble Space Telescope and future observatories like the James Webb Space Telescope, which will provide higher resolution images and spectra, further validating or challenging our current theories. In summary, historical theories and predictions have been instrumental in guiding our understanding of stellar physics, even as observational challenges persist, highlighting the ongoing interplay between theory and observation in astrophysics.
Physical Characteristics of Stars
Stars, the celestial bodies that illuminate our universe, exhibit a wide range of physical characteristics that define their nature and behavior. Understanding these characteristics is crucial for astronomers to classify and study stars effectively. This article delves into three key aspects of stellar physics: Surface Temperature and Color, Size and Mass Variations, and Atmospheric Composition and Activity. Each of these factors plays a significant role in determining the star's life cycle, energy output, and overall impact on its surroundings. Surface Temperature and Color, for instance, are closely linked and influence the star's spectral type, which in turn affects its luminosity and evolutionary path. Size and Mass Variations distinguish stars into different categories such as main-sequence stars, red giants, and white dwarfs, each with unique properties. Atmospheric Composition and Activity reveal insights into the star's chemical makeup and dynamic processes like stellar winds and magnetic fields. By exploring these physical characteristics, we gain a deeper understanding of the diverse universe of stars. Let us begin by examining the relationship between Surface Temperature and Color, a fundamental aspect that sets the stage for understanding the broader spectrum of stellar attributes.
Surface Temperature and Color
Surface temperature and color are two closely intertwined physical characteristics of stars that provide valuable insights into their nature. The surface temperature of a star, often referred to as its effective temperature, is a measure of the temperature at the photosphere—the layer from which light is emitted. This temperature ranges from about 3,000 Kelvin for red dwarf stars to over 50,000 Kelvin for blue giant stars. The color of a star is directly related to its surface temperature due to the principles of blackbody radiation. According to Wien's displacement law, hotter stars emit more light in the blue and ultraviolet parts of the spectrum, appearing blue or white, while cooler stars emit more light in the red and infrared parts, appearing red or orange. For instance, red giant stars have surface temperatures around 3,000 to 4,000 Kelvin, which is why they appear red. On the other hand, blue-white stars like those in the O-type category have surface temperatures exceeding 30,000 Kelvin, making them appear intensely blue. The Sun, with a surface temperature of about 5,500 Kelvin, appears yellow to our eyes because it emits most of its light in the yellow part of the visible spectrum. Understanding the relationship between surface temperature and color is crucial for astronomers studying stellar properties. By observing the color of a star, astronomers can infer its surface temperature and subsequently derive other important characteristics such as luminosity and mass. This method is particularly useful when direct measurements are not feasible due to distance or other observational limitations. Moreover, the color-temperature relationship helps in classifying stars into different spectral types. The Harvard Spectral Classification Scheme categorizes stars into O, B, A, F, G, K, and M types based on their surface temperatures and corresponding colors. This classification system is fundamental in understanding stellar evolution and the life cycles of stars. In summary, the surface temperature and color of a star are intricately linked and serve as key indicators of its physical properties. By analyzing these characteristics, astronomers gain valuable insights into the nature and behavior of stars, enabling a deeper understanding of the universe's stellar population.
Size and Mass Variations
Stars exhibit a wide range of sizes and masses, which are fundamental physical characteristics that influence their lifecycles, luminosities, and eventual fates. The size of a star is typically measured in terms of its radius, which can vary from about 10% to over 1,000 times the radius of the Sun. For instance, red dwarf stars are the smallest, with radii roughly 10-50% that of the Sun, while red giant stars can swell to sizes 100 times larger than our Sun. The mass of a star, on the other hand, is usually expressed in solar masses (M⊙), where one solar mass is the mass of our Sun. Stellar masses range from about 0.1 M⊙ for the smallest red dwarfs to over 100 M⊙ for the most massive stars known as O-type or blue giants. The relationship between size and mass is not straightforward; it depends on the star's evolutionary stage and composition. Main-sequence stars, which are fusing hydrogen into helium in their cores, follow a mass-radius relation where more massive stars are generally larger but not proportionally so. For example, a star with twice the mass of the Sun will have a radius about 1.2 times larger. However, as stars evolve off the main sequence and become red giants or white dwarfs, their sizes can change dramatically without corresponding changes in mass. Mass is a critical determinant of a star's lifecycle. Low-mass stars like red dwarfs live for billions of years due to their slow nuclear fusion rates, while high-mass stars burn through their fuel rapidly and have lifetimes of only a few million years. These massive stars end their lives in spectacular supernovae explosions, dispersing heavy elements into space that eventually form new stars and planets. In contrast, low-mass stars like our Sun will exhaust their fuel more slowly and expand into red giants before shedding their outer layers to form white dwarfs. The variations in size and mass also affect the surface temperature and luminosity of stars. More massive stars are hotter and more luminous because they have higher core pressures and temperatures that drive more vigorous nuclear reactions. This is why O-type stars are among the brightest objects in the galaxy despite their relatively short lifetimes. Conversely, low-mass stars are cooler and less luminous but can sustain life for extended periods. Understanding these variations in size and mass is crucial for astrophysicists studying star formation, stellar evolution, and the overall structure of galaxies. By analyzing these physical characteristics through observations and simulations, scientists can gain insights into how stars contribute to cosmic processes such as element synthesis, planetary formation, and the dynamics of stellar clusters and galaxies. In summary, the diverse range of sizes and masses among stars underpins much of what we understand about their behavior, evolution, and impact on the universe. From tiny red dwarfs to massive blue giants, each type of star plays a unique role in shaping our cosmic landscape.
Atmospheric Composition and Activity
Atmospheric composition and activity are crucial aspects of understanding the physical characteristics of stars, particularly when examining what a star looks like up close. The atmosphere of a star is the outer layer where light is emitted and absorbed, influencing its observable properties. The primary components of a star's atmosphere include hydrogen, helium, and trace amounts of heavier elements such as oxygen, carbon, and iron. These elements interact through various processes, including ionization, excitation, and recombination, which affect the star's spectral lines and overall luminosity. The activity within a star's atmosphere can be quite dynamic. Stellar atmospheres are subject to convective motions, where hot material rises to the surface while cooler material sinks, creating granular patterns visible in high-resolution images of stars like the Sun. Magnetic fields also play a significant role, driving phenomena such as sunspots and solar flares in our Sun and analogous activities in other stars. These magnetic fields can lead to the formation of coronae, the outermost layers of the atmosphere that are millions of degrees hotter than the surface. In addition to these internal processes, external factors such as stellar winds and mass loss can alter the atmospheric composition over time. For example, red giant stars shed significant portions of their outer layers into space, enriching the interstellar medium with heavy elements synthesized during their lifetimes. This mass loss can be observed in the form of planetary nebulae or other circumstellar structures. Observing these atmospheric activities up close is challenging due to the immense distances between Earth and other stars. However, advanced telescopes and spectrographic techniques allow astronomers to infer detailed information about stellar atmospheres. By analyzing spectral lines and their shifts (Doppler shifts), scientists can determine a star's temperature, chemical composition, rotation rate, and even the presence of exoplanets. Understanding the atmospheric composition and activity of stars is essential for broader astrophysical studies. It helps in classifying stars into different types based on their spectral characteristics (e.g., O, B, A, F, G, K, M), which in turn relates to their mass, age, and evolutionary stage. This knowledge also aids in understanding stellar evolution processes and how stars contribute to the chemical enrichment of galaxies. In summary, the atmospheric composition and activity of stars are vital components of their physical characteristics. These factors influence how we observe and classify stars, providing insights into their internal dynamics and evolutionary histories. By studying these aspects up close through advanced observational techniques, astronomers gain a deeper understanding of what makes each star unique and how they fit into the broader cosmic landscape.
Up-Close Observations: What We Know So Far
Up-Close Observations: What We Know So Far delves into the fascinating realm of astronomical research, where advanced technologies and meticulous studies have significantly enhanced our understanding of celestial bodies. This article explores three key areas that have revolutionized our knowledge: High-Resolution Imaging Techniques, Stellar Surface Features and Patterns, and Case Studies: Close-Up Views of Specific Stars. By leveraging high-resolution imaging techniques, scientists can now capture detailed images of distant stars and planets, revealing intricate details that were previously invisible. These techniques have enabled the identification of stellar surface features and patterns, providing insights into the internal dynamics and evolutionary stages of stars. Furthermore, case studies of close-up views of specific stars offer a deeper understanding of their unique characteristics and behaviors. As we transition into the specifics, we will first examine the High-Resolution Imaging Techniques that have made these groundbreaking observations possible.
High-Resolution Imaging Techniques
High-resolution imaging techniques have revolutionized our ability to observe celestial objects, including stars, with unprecedented detail. These advanced methods allow astronomers to capture images that reveal intricate structures and phenomena that were previously invisible. One of the key techniques is **adaptive optics**, which compensates for the distortion caused by Earth's atmosphere, enabling ground-based telescopes to achieve resolutions comparable to those of space-based telescopes. This technology uses deformable mirrors and sophisticated algorithms to correct for atmospheric interference, resulting in sharper images of stars and other celestial bodies. Another crucial technique is **interferometry**, where multiple telescopes are combined to form a virtual telescope with a diameter equal to the distance between the individual telescopes. This method significantly enhances resolution by allowing astronomers to study the fine details of stellar surfaces, such as sunspots on the Sun or convective patterns on other stars. **Space telescopes**, like the Hubble Space Telescope and the James Webb Space Telescope, also play a pivotal role in high-resolution imaging. Operating above the Earth's atmosphere, these telescopes can capture images in various wavelengths, including infrared and ultraviolet, which are blocked by atmospheric gases. This capability provides insights into the composition and temperature of stellar atmospheres. **Spectroscopy** is another powerful tool that complements high-resolution imaging. By analyzing the light spectrum emitted by stars, scientists can determine their chemical composition, temperature, and even their motion relative to Earth. When combined with high-resolution imaging, spectroscopy offers a comprehensive view of stellar properties, enabling detailed studies of stellar evolution and behavior. **Lucky imaging** is another innovative approach that leverages short exposure times and advanced processing algorithms to select and combine the sharpest frames from a series of images. This technique is particularly useful for ground-based telescopes, as it mitigates the effects of atmospheric turbulence, allowing for higher resolution images than would otherwise be possible. In addition, **coronagraphy** is used to study the immediate surroundings of stars by blocking the direct light from the star itself. This allows astronomers to observe faint objects like exoplanets or protoplanetary disks that would otherwise be overwhelmed by the star's brightness. By combining these high-resolution imaging techniques with advanced data processing and analysis, scientists can gain profound insights into the structure, dynamics, and evolution of stars, providing a clearer understanding of what stars look like up close. These advancements have transformed our understanding of stellar astronomy, enabling us to explore the universe with greater precision and detail than ever before.
Stellar Surface Features and Patterns
When observing stellar surfaces up close, astronomers uncover a myriad of fascinating features and patterns that reveal the intricate dynamics of these celestial bodies. One of the most striking aspects is the presence of **granules**, which are convective cells that form due to the transport of heat from the star's interior to its surface. These granules appear as bright, irregularly shaped patches surrounded by darker lanes, known as intergranular lanes, where cooler material sinks back into the star. On our Sun, these granules are about 1,000 kilometers in diameter and have lifetimes of around 10 minutes, though they can vary significantly in size and duration on other stars. Another key feature is **sunspots**, which are cooler regions on the stellar surface caused by intense magnetic fields that inhibit convection. These spots are darker than the surrounding areas because they are cooler, typically by about 1,500 degrees Celsius compared to the rest of the surface. Sunspots can be massive, sometimes covering areas larger than Earth itself, and they play a crucial role in understanding stellar activity and magnetic cycles. **Faculae** are bright regions often found near sunspots or in areas of strong magnetic activity. These areas are hotter than the surrounding surface and appear brighter due to the concentration of magnetic flux, which inhibits convection and allows more heat to escape. Faculae are particularly visible during solar eclipses or when viewed through specialized filters that highlight their contrast against the cooler background. The **chromosphere**, a layer just above the photosphere (the visible surface), also exhibits unique patterns. Here, you find **spicules**, which are narrow, jet-like features that shoot up from the surface at speeds of up to 20 kilometers per second. These spicules are thought to be driven by magnetic reconnection events and play a significant role in heating the chromosphere and corona. The **corona**, the outermost layer of a star's atmosphere, is another region of interest with its own set of features. The corona is much hotter than the surface below it, with temperatures reaching millions of degrees Celsius. This layer is visible during total solar eclipses as a glowing halo around the Sun and is characterized by **coronal loops** and **prominences**. Coronal loops are magnetic field lines that arc above the surface, while prominences are large, cloud-like structures that can erupt as coronal mass ejections (CMEs), affecting space weather. In addition to these specific features, stellar surfaces also exhibit broader patterns such as **stellar rotation** and **differential rotation**. The rotation of a star can cause its equator to bulge outward due to centrifugal force, while differential rotation means that different latitudes rotate at different speeds. This differential rotation can lead to complex magnetic field configurations and enhanced activity at certain latitudes. Understanding these surface features and patterns is crucial for gaining insights into the internal dynamics and evolutionary stages of stars. By studying these phenomena up close through advanced telescopes and space missions, scientists can better comprehend how stars like our Sun generate their energy and how they influence their surrounding environments. This knowledge not only enhances our understanding of stellar physics but also has implications for understanding planetary habitability and the broader context of our place in the universe.
Case Studies: Close-Up Views of Specific Stars
**Case Studies: Close-Up Views of Specific Stars** When delving into the intricacies of stellar anatomy, case studies of specific stars provide invaluable insights. These detailed examinations allow astronomers to dissect the characteristics, behaviors, and life cycles of individual stars, offering a nuanced understanding that general theories alone cannot provide. For instance, the study of Betelgeuse, a red supergiant in the constellation Orion, has revealed significant details about stellar evolution. Observations have shown that Betelgeuse is nearing the end of its life cycle and is expected to explode as a supernova in the near future, providing scientists with a unique opportunity to study the final stages of a massive star's life. Similarly, the analysis of Proxima Centauri, the closest star to the Sun, has shed light on the properties of small, cool stars known as red dwarfs. Proxima Centauri's low mass and surface temperature make it an ideal subject for studying the dynamics of planetary systems around such stars, particularly in the context of exoplanet hunting and the search for potentially habitable worlds. Another notable example is Sirius, the brightest star in the night sky, which has been extensively studied for its binary nature and its impact on our understanding of stellar companionship and orbital dynamics. The case study of R136a1, a massive star in the Large Magellanic Cloud, highlights the extreme conditions found in some stellar environments. With a mass over 265 times that of the Sun, R136a1 is one of the most massive stars known, offering insights into how such behemoths form and influence their surroundings through intense radiation and strong stellar winds. These close-up views not only enrich our knowledge of individual stars but also contribute to a broader understanding of stellar populations and their roles within galaxies. By focusing on these specific stars, astronomers can gather precise data on their luminosity, temperature, composition, and other key parameters. This detailed information helps in refining models of stellar structure and evolution, ultimately enhancing our comprehension of how stars are born, live, and die. Moreover, these case studies often serve as benchmarks for comparing other stars within similar categories, allowing for a more systematic approach to stellar classification and research. In essence, these close-up views of specific stars act as windows into the diverse universe of stellar phenomena, each offering unique lessons that collectively enrich our understanding of the cosmos.