What Is Rna Polymerase
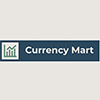
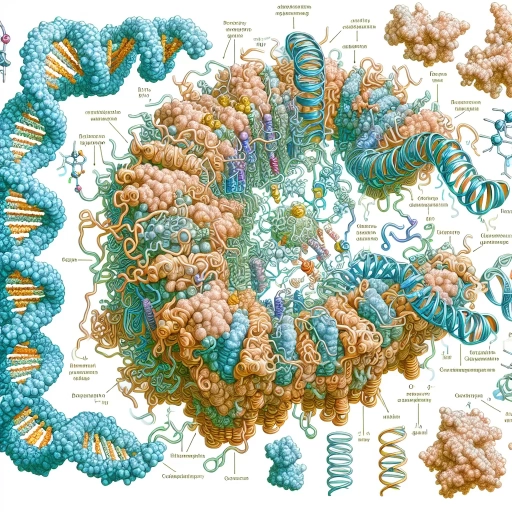
RNA polymerase is a crucial enzyme at the heart of cellular transcription, the process by which genetic information encoded in DNA is transcribed into RNA. This enzyme plays a pivotal role in synthesizing RNA from a DNA template, enabling the translation of genetic instructions into proteins and other essential cellular functions. Understanding RNA polymerase involves delving into its structure and mechanism, which reveal how it accurately reads DNA sequences and assembles nucleotides into a complementary RNA strand. Additionally, there are various types and variations of RNA polymerase, each specialized for different roles within the cell, such as prokaryotic and eukaryotic RNA polymerases. This article will explore these facets in detail, beginning with an introduction to RNA polymerase, followed by an examination of its structure and mechanism, and concluding with a discussion on the diverse types and variations of this vital enzyme. Let us start by introducing RNA polymerase, the foundational component of transcriptional processes.
Introduction to RNA Polymerase
RNA polymerase is a crucial enzyme at the heart of cellular transcription, playing a pivotal role in the synthesis of RNA from DNA templates. This enzyme is fundamental to the expression of genetic information, facilitating the translation of genes into proteins that perform a wide array of cellular functions. To fully understand RNA polymerase, it is essential to delve into its definition and function, which will be explored in detail. However, its significance extends beyond its biochemical role; it has a rich historical context that highlights its discovery and the impact it has had on our understanding of molecular biology. The historical discovery of RNA polymerase marked a significant milestone in the field, shedding light on the mechanisms of gene expression and opening new avenues for research. Additionally, RNA polymerase's role in various cellular processes, such as transcription initiation, elongation, and termination, underscores its central importance in maintaining cellular homeostasis and responding to environmental cues. By examining these aspects, we can gain a comprehensive understanding of RNA polymerase's multifaceted nature and its indispensable role in cellular biology. Let us begin by exploring the definition and function of RNA polymerase, which forms the foundation of its critical biological activities.
Definition and Function
RNA polymerase is a crucial enzyme that plays a pivotal role in the process of transcription, which is the first step in the central dogma of molecular biology. **Definition and Function** of RNA polymerase are intricately linked, as this enzyme is responsible for synthesizing RNA from a DNA template. At its core, RNA polymerase is a molecular machine that unwinds the double helix structure of DNA, reads the template strand, and matches the incoming nucleotides to the base pairing rules (A-T and G-C), thereby elongating the RNA chain. The function of RNA polymerase is multifaceted and highly regulated. It initiates transcription by binding to specific DNA sequences known as promoters, which are located upstream of the genes to be transcribed. Once bound, the enzyme undergoes a conformational change that allows it to unwind the DNA double helix, creating a transcription bubble. Within this bubble, the template strand of DNA is read in a 3' to 5' direction, while the nascent RNA strand is synthesized in a 5' to 3' direction. This process involves the incorporation of nucleotides into the growing RNA chain through phosphodiester bond formation. RNA polymerase also possesses proofreading and editing capabilities, ensuring that the synthesized RNA is accurate and free from errors. This is particularly important because errors in transcription can lead to aberrant gene expression and potentially harmful cellular outcomes. Additionally, RNA polymerase interacts with various transcription factors and regulatory proteins that modulate its activity, allowing for precise control over gene expression in response to cellular signals and environmental changes. In prokaryotic cells, there is a single type of RNA polymerase responsible for transcribing all types of RNA, including messenger RNA (mRNA), transfer RNA (tRNA), and ribosomal RNA (rRNA). In contrast, eukaryotic cells have multiple forms of RNA polymerase, each specialized for different types of RNA synthesis: RNA polymerase I for rRNA, RNA polymerase II for mRNA and most small nuclear RNAs, and RNA polymerase III for tRNA and other small RNAs. The efficiency and specificity of RNA polymerase are further enhanced by its ability to form complexes with other proteins and factors that facilitate initiation, elongation, and termination phases of transcription. For instance, in eukaryotes, the general transcription factors (GTFs) such as TFIID, TFIIA, TFIIB, TFIIF, TFIIE, and TFIIH work in concert with RNA polymerase II to ensure accurate and regulated transcription. In summary, the definition and function of RNA polymerase are deeply intertwined, reflecting its critical role in converting genetic information stored in DNA into RNA molecules that can be translated into proteins or perform other cellular functions. Its precision, regulatory complexity, and specialized forms underscore its importance as a central enzyme in the process of gene expression. Understanding RNA polymerase is essential for grasping the fundamental mechanisms of cellular biology and has significant implications for fields such as genetics, molecular biology, and biotechnology.
Historical Discovery and Significance
The discovery of RNA polymerase is a pivotal moment in the history of molecular biology, marking a significant milestone in our understanding of gene expression and the central dogma of molecular biology. This enzyme, first identified in the 1960s by researchers such as Samuel Weiss and Jerard Hurwitz, plays a crucial role in the transcription process, where DNA is transcribed into RNA. The historical significance of this discovery lies in its profound impact on our comprehension of how genetic information is translated from DNA to proteins. Prior to the identification of RNA polymerase, scientists were aware that DNA served as the template for RNA synthesis, but the mechanism by which this occurred was unknown. The isolation and characterization of RNA polymerase by Weiss and Hurwitz in 1960 provided the missing link, revealing that this enzyme is responsible for unwinding the DNA double helix and synthesizing a complementary RNA strand. This breakthrough not only elucidated a key step in gene expression but also opened up new avenues for research into the regulation of gene transcription. The significance of RNA polymerase extends beyond its role in transcription. It has become a cornerstone in understanding various biological processes, including development, cell differentiation, and disease mechanisms. For instance, dysregulation of RNA polymerase activity has been implicated in several diseases, such as cancer and neurodegenerative disorders. Moreover, the study of RNA polymerase has led to the development of therapeutic strategies, including antibiotics that target bacterial RNA polymerase to inhibit bacterial growth. From a historical perspective, the discovery of RNA polymerase was part of a broader era of scientific inquiry that transformed our understanding of molecular biology. It followed closely on the heels of other landmark discoveries, such as the structure of DNA by James Watson and Francis Crick in 1953 and the deciphering of the genetic code in the early 1960s. Together, these findings formed a cohesive narrative about how genetic information is encoded, transcribed, and translated into proteins. In contemporary research, RNA polymerase remains a focal point for studies aimed at understanding the intricacies of gene regulation. Advances in structural biology have provided detailed insights into the architecture and function of RNA polymerase, enabling researchers to design inhibitors and modulators that can manipulate its activity. This has far-reaching implications for biotechnology and medicine, where precise control over gene expression is crucial. In conclusion, the historical discovery of RNA polymerase is a testament to the power of scientific inquiry and its ability to illuminate fundamental biological processes. As we continue to explore the complexities of gene expression, this enzyme remains at the forefront, offering insights into both basic biological mechanisms and potential therapeutic interventions. Its significance underscores the importance of continued research into the molecular machinery that underpins life itself.
Role in Cellular Processes
RNA polymerase plays a pivotal role in cellular processes, particularly in the transcription phase of gene expression. This enzyme is responsible for synthesizing RNA from a DNA template, a process that is fundamental to the translation of genetic information into proteins. At the heart of transcription, RNA polymerase unwinds the double helix structure of DNA, allowing it to read the template strand and match the incoming nucleotides to the base pairing rules. This precise mechanism ensures that the genetic code is accurately transcribed into a complementary RNA molecule. In eukaryotic cells, there are three main types of RNA polymerase: RNA polymerase I, II, and III. Each type has specific roles in transcribing different types of RNA. RNA polymerase I is primarily involved in the synthesis of ribosomal RNA (rRNA), which forms the structural components of ribosomes. RNA polymerase II is crucial for transcribing messenger RNA (mRNA) and most small nuclear RNAs (snRNAs), which are essential for splicing mRNA. RNA polymerase III transcribes transfer RNA (tRNA) and other small RNAs. The activity of RNA polymerase is tightly regulated to ensure that gene expression is coordinated with cellular needs. Various transcription factors and regulatory elements such as enhancers and promoters modulate the binding of RNA polymerase to the DNA template. Once bound, the enzyme undergoes a series of conformational changes that facilitate the initiation of transcription. During elongation, RNA polymerase moves along the DNA template, adding nucleotides to the growing RNA chain. Finally, termination signals on the DNA template trigger the release of the newly synthesized RNA molecule. In addition to its central role in transcription, RNA polymerase also interacts with other cellular machinery to ensure efficient gene expression. For instance, it coordinates with chromatin remodeling complexes to access DNA sequences that are compacted into chromatin. Furthermore, RNA polymerase can recruit other enzymes and factors necessary for post-transcriptional modifications, such as capping and splicing of mRNA. Dysregulation of RNA polymerase activity has been implicated in various diseases, including cancer and genetic disorders. Mutations in genes encoding subunits of RNA polymerase or its regulatory factors can lead to aberrant gene expression patterns, contributing to disease pathogenesis. Understanding the mechanisms and regulation of RNA polymerase is thus crucial for developing therapeutic strategies aimed at correcting these imbalances. In summary, RNA polymerase is a multifaceted enzyme that orchestrates the transcriptional process with precision and accuracy. Its role extends beyond mere RNA synthesis; it integrates with broader cellular processes to ensure the faithful translation of genetic information into functional proteins. The intricate regulation and diverse functions of RNA polymerase underscore its importance as a cornerstone of cellular biology.
Structure and Mechanism of RNA Polymerase
RNA polymerase, the central enzyme in the transcription process, is a complex molecular machine that orchestrates the synthesis of RNA from a DNA template. Understanding its structure and mechanism is crucial for grasping how genetic information is transcribed into functional RNA molecules. This article delves into the intricate details of RNA polymerase, focusing on three key aspects: Subunit Composition and Assembly, Transcription Initiation and Elongation, and Termination Process and Regulation. Firstly, the subunit composition and assembly of RNA polymerase are essential for its functionality. The enzyme is composed of multiple subunits that come together to form a functional complex, each subunit playing a specific role in the transcription process. Secondly, transcription initiation and elongation involve the binding of RNA polymerase to the DNA template, unwinding of the DNA double helix, and the synthesis of the RNA strand. This phase is critical for ensuring accurate and efficient transcription. Finally, the termination process and regulation mechanisms ensure that transcription is properly concluded and that the RNA product is correctly processed and modified. By examining these components, we can gain a comprehensive understanding of how RNA polymerase operates at the molecular level. Let us begin by exploring the subunit composition and assembly of RNA polymerase, which lays the foundation for its overall structure and function.
Subunit Composition and Assembly
RNA polymerase, the central enzyme in the transcription process, is a complex molecular machine composed of multiple subunits that work in concert to synthesize RNA from a DNA template. The subunit composition and assembly of RNA polymerase are crucial for its function and vary slightly between different types of organisms. In prokaryotes, such as bacteria, RNA polymerase is primarily composed of five subunits: two alpha (α) subunits, one beta (β) subunit, one beta-prime (β') subunit, and one omega (ω) subunit. The core enzyme, which consists of the β, β', and two α subunits, is responsible for the catalytic activity and processivity of RNA synthesis. The sigma (σ) factor, which associates with the core enzyme to form the holoenzyme, plays a critical role in promoter recognition and initiation of transcription. The assembly of these subunits is highly regulated and ensures precise recognition of promoter sequences and efficient transcription initiation. In eukaryotes, the structure of RNA polymerase is more complex and diverse. There are three main types of RNA polymerases in eukaryotic cells: RNA polymerase I, II, and III. Each type has a distinct subunit composition tailored to its specific function. For example, RNA polymerase II, which is responsible for transcribing protein-coding genes, consists of 12 subunits (Rpb1-12). The largest subunit, Rpb1, contains the active site where nucleotide addition occurs during transcription elongation. The assembly of these subunits is tightly regulated and involves various accessory proteins that facilitate the recruitment of RNA polymerase to specific promoters and the initiation of transcription. The assembly process itself is a highly coordinated event involving multiple steps. In prokaryotes, the core enzyme assembles first, followed by the binding of the sigma factor to form the holoenzyme. In eukaryotes, the assembly involves a series of interactions between the various subunits and accessory factors that ensure proper positioning and function. This precise assembly is essential for the enzyme's ability to recognize and bind to specific DNA sequences, unwind the DNA double helix, and accurately synthesize RNA. Understanding the subunit composition and assembly of RNA polymerase provides valuable insights into its mechanism of action. It highlights the intricate molecular interactions required for efficient transcription and underscores the importance of structural integrity in maintaining enzymatic function. Moreover, this knowledge has significant implications for understanding transcriptional regulation and the development of therapeutic strategies targeting RNA polymerase in diseases such as cancer and viral infections. By elucidating the detailed architecture and assembly process of RNA polymerase, researchers can better appreciate the complexity and precision of this fundamental biological process.
Transcription Initiation and Elongation
Transcription initiation and elongation are crucial steps in the process of RNA synthesis, mediated by the enzyme RNA polymerase. **Transcription initiation** begins with the binding of RNA polymerase to a specific DNA sequence known as the promoter region. This binding is facilitated by transcription factors that help position the polymerase correctly. Once bound, the polymerase unwinds the double helix, creating a transcription bubble where the template strand is exposed. The process is further regulated by the melting of the DNA double helix at the promoter region, allowing the polymerase to start synthesizing RNA from a specific start site. This initial phase is highly regulated and involves various checkpoints to ensure accurate and controlled transcription. Following initiation, **transcription elongation** commences as RNA polymerase moves along the template DNA strand, synthesizing a complementary RNA molecule. During elongation, the polymerase reads the template strand in a 5' to 3' direction, adding nucleotides to the growing RNA chain according to base pairing rules. The elongation phase involves continuous unwinding of DNA ahead of the polymerase and rewinding behind it, maintaining a stable transcription bubble. This process is remarkably efficient, with some RNA polymerases capable of synthesizing thousands of nucleotides per minute. However, it is not without challenges; the polymerase must navigate obstacles such as DNA-bound proteins and secondary structures, often pausing or backtracking to correct errors. The structure of RNA polymerase plays a pivotal role in both initiation and elongation. The enzyme's active site, located within its central cleft, is where nucleotide addition occurs. The polymerase also features a clamp that opens to allow DNA binding and closes to secure the template during elongation. Additionally, the enzyme's proofreading and editing mechanisms ensure high fidelity in RNA synthesis by correcting mismatched bases. These structural elements work in concert to facilitate smooth and accurate transcription, highlighting the intricate interplay between the enzyme's architecture and its functional capabilities. In summary, transcription initiation and elongation are complex processes that rely on the precise interaction between RNA polymerase and DNA. The initiation phase sets the stage for RNA synthesis by positioning the polymerase correctly and unwinding the DNA double helix. During elongation, the polymerase efficiently synthesizes RNA while navigating potential obstacles. The structure of RNA polymerase, with its specialized domains and active site, is essential for these processes, underscoring the enzyme's critical role in gene expression. Understanding these mechanisms provides valuable insights into how cells regulate and execute the fundamental process of transcription.
Termination Process and Regulation
The termination process of RNA transcription, a crucial step in the lifecycle of RNA polymerase, is meticulously regulated to ensure precise and efficient gene expression. This process involves the coordinated action of various factors and mechanisms that signal the enzyme to halt transcription and release the newly synthesized RNA transcript. In prokaryotes, termination can occur through two primary mechanisms: intrinsic (rho-independent) and extrinsic (rho-dependent) termination. Intrinsic termination involves the formation of a hairpin loop in the nascent RNA transcript followed by a U-rich region, which causes the RNA-DNA hybrid to destabilize, leading to the dissociation of RNA polymerase from the DNA template. This mechanism relies on the secondary structure of the RNA itself and does not require additional proteins. In contrast, extrinsic termination involves the action of the rho protein, which binds to specific sequences on the nascent RNA transcript known as rho utilization (rut) sites. Rho then moves along the RNA, using its helicase activity to unwind the RNA-DNA hybrid, thereby causing RNA polymerase to terminate transcription. This mechanism is particularly important for ensuring that transcription is terminated efficiently and accurately, especially in cases where intrinsic termination signals are weak or absent. In eukaryotes, the termination process is more complex and involves multiple factors and pathways. One key mechanism involves the cleavage and polyadenylation of the pre-mRNA transcript, which signals the end of transcription. This process is mediated by a set of proteins that recognize specific sequences near the 3' end of the gene and cleave the RNA, followed by the addition of a poly(A) tail. Another mechanism in eukaryotes involves the use of termination factors such as TTF2 and Pcf11, which interact with RNA polymerase II to facilitate its release from the DNA template. Regulation of termination is critical for maintaining genomic integrity and preventing aberrant gene expression. Dysregulation in termination can lead to read-through transcription, where RNA polymerase fails to stop at the intended termination site, potentially resulting in the production of aberrant transcripts that could interfere with cellular function. Therefore, precise regulation of termination ensures that genes are expressed correctly and that cellular resources are allocated efficiently. Understanding the termination process and its regulation is essential for appreciating the intricate mechanisms that govern gene expression. The structure and mechanism of RNA polymerase itself play a pivotal role in this process, as the enzyme must be capable of recognizing termination signals and responding appropriately to ensure accurate and efficient transcription. The interplay between RNA polymerase and termination factors highlights the complexity and sophistication of the transcriptional machinery, underscoring the importance of continued research into these processes to elucidate their roles in both normal cellular function and disease states.
Types and Variations of RNA Polymerase
RNA polymerase, the central enzyme in the transcription process, exhibits a remarkable diversity in its types and variations, each tailored to specific cellular environments and functions. This article delves into the intricate world of RNA polymerases, exploring three key aspects that highlight their complexity and specialization. First, we will examine the distinctions between prokaryotic and eukaryotic RNA polymerases, uncovering how these differences reflect the unique transcriptional needs of these cellular types. Next, we will explore specialized forms of RNA polymerase found in mitochondria and chloroplasts, which have evolved to manage the transcriptional demands of these organelles. Finally, we will discuss the variations in promoter recognition and the role of transcription factors, shedding light on how these elements influence the initiation and regulation of transcription. By understanding these facets, we gain a deeper appreciation for the versatility and precision of RNA polymerase in orchestrating gene expression across diverse biological contexts. Let us begin by comparing the fundamental differences between prokaryotic and eukaryotic RNA polymerases, a distinction that sets the stage for understanding the broader spectrum of RNA polymerase functions.
Prokaryotic vs. Eukaryotic RNA Polymerases
RNA polymerases are the enzymes responsible for synthesizing RNA from a DNA template, a process crucial for gene expression. When comparing prokaryotic and eukaryotic RNA polymerases, several key differences emerge that reflect the distinct cellular environments and regulatory needs of these organisms. **Prokaryotic RNA Polymerase:** In prokaryotes, such as bacteria, RNA polymerase is a single enzyme that transcribes all types of RNA, including messenger RNA (mRNA), transfer RNA (tRNA), and ribosomal RNA (rRNA). This enzyme is composed of five subunits: two alpha subunits, one beta subunit, one beta-prime subunit, and one omega subunit. The core enzyme, consisting of the beta, beta-prime, and two alpha subunits, is capable of elongation but requires the sigma factor for promoter recognition and initiation. Sigma factors are interchangeable and allow the polymerase to recognize different promoter sequences, thereby regulating gene expression in response to environmental changes. **Eukaryotic RNA Polymerase:** In contrast, eukaryotes have multiple types of RNA polymerases, each specialized for different types of RNA synthesis. There are three main types: RNA Polymerase I, II, and III. **RNA Polymerase I** is responsible for transcribing the large ribosomal RNA precursor (45S rRNA) in the nucleolus. **RNA Polymerase II** transcribes protein-coding genes to produce mRNA and is also involved in the synthesis of some small nuclear RNAs (snRNAs) and microRNAs (miRNAs). This polymerase is highly regulated and requires a complex assembly of general transcription factors (GTFs) for initiation. **RNA Polymerase III** transcribes small RNAs, including tRNAs, 5S rRNA, and some snRNAs. **Key Differences:** 1. **Structure and Composition:** Prokaryotic RNA polymerase is a single enzyme with fewer subunits compared to the multiple, more complex eukaryotic enzymes. 2. **Promoter Recognition:** Prokaryotic RNA polymerase uses sigma factors for promoter recognition, while eukaryotic RNA polymerases rely on specific DNA sequences and a variety of transcription factors. 3. **Regulation:** Eukaryotic transcription is more tightly regulated due to the presence of enhancers, silencers, and other cis-regulatory elements that interact with transcription factors. 4. **Transcription Initiation:** The initiation process in eukaryotes involves a pre-initiation complex (PIC) formation which is more complex compared to the simpler sigma factor-based system in prokaryotes. 5. **Post-transcriptional Processing:** Eukaryotic mRNAs undergo extensive post-transcriptional processing, including splicing, capping, and polyadenylation, which is not seen in prokaryotes. These differences highlight the evolutionary adaptations that have allowed eukaryotes to achieve greater complexity and regulation in gene expression compared to prokaryotes. Understanding these variations is essential for appreciating the intricate mechanisms of transcription and its role in cellular function across different domains of life.
Specialized Forms: Mitochondrial and Chloroplast
RNA polymerase, the enzyme responsible for synthesizing RNA from a DNA template, exhibits a variety of forms tailored to specific cellular environments and functions. Among these specialized forms are those found in mitochondria and chloroplasts, organelles that have their own distinct genetic material and transcriptional machinery. **Mitochondrial RNA Polymerase:** Mitochondrial RNA polymerase, often referred to as POLRMT (mitochondrial RNA polymerase), is uniquely adapted to transcribe the mitochondrial genome. Unlike the nuclear RNA polymerases, which are multi-subunit enzymes, POLRMT is a single-subunit enzyme that shares structural and functional similarities with the T7 RNA polymerase of bacteriophages. This enzyme is essential for the transcription of mitochondrial DNA (mtDNA), which encodes genes crucial for mitochondrial function, including those involved in the electron transport chain and oxidative phosphorylation. The specificity of POLRMT ensures that mitochondrial transcription is regulated independently of nuclear transcription, allowing mitochondria to maintain their own genetic autonomy. **Chloroplast RNA Polymerase:** Chloroplasts, the organelles responsible for photosynthesis in plants and some algae, contain their own RNA polymerases. There are two main types of chloroplast RNA polymerases: the plastid-encoded RNA polymerase (PEP) and the nuclear-encoded RNA polymerase (NEP). PEP is a multi-subunit enzyme similar to bacterial RNA polymerase and is responsible for transcribing genes involved in photosynthesis and other essential chloroplast functions. NEP, on the other hand, is a single-subunit enzyme that transcribes housekeeping genes and other non-photosynthetic genes within the chloroplast genome. The dual system of PEP and NEP allows for complex regulation of chloroplast gene expression, ensuring that photosynthetic activity is finely tuned to environmental conditions. **Functional Significance:** The specialized forms of RNA polymerase in mitochondria and chloroplasts underscore the evolutionary adaptations that have allowed these organelles to maintain functional independence while still being integrated into the broader cellular context. These enzymes play critical roles in ensuring that the unique genetic material within these organelles is transcribed accurately and efficiently. For instance, mitochondrial RNA polymerase must contend with the high mutation rate of mtDNA, while chloroplast RNA polymerases must coordinate gene expression with light availability and other environmental factors. The distinct properties of these enzymes highlight the intricate mechanisms that govern organelle-specific transcription and underscore the importance of understanding these processes for insights into cellular biology and potential therapeutic applications. In summary, the specialized forms of RNA polymerase found in mitochondria and chloroplasts are vital components of organelle-specific transcriptional machinery. These enzymes have evolved to meet the unique demands of their respective environments, ensuring that the genetic material within these organelles is transcribed with precision and efficiency. Their study not only deepens our understanding of cellular biology but also opens avenues for exploring new therapeutic strategies and understanding diseases related to mitochondrial and chloroplast dysfunction.
Differences in Promoter Recognition and Transcription Factors
The process of transcription, which involves the synthesis of RNA from a DNA template, is intricately regulated by the interplay between RNA polymerase and various transcription factors. One of the critical steps in this process is promoter recognition, where RNA polymerase must accurately identify and bind to specific DNA sequences known as promoters. Here, differences in promoter recognition and the involvement of transcription factors play pivotal roles in determining the efficiency and specificity of transcription. RNA polymerase, the central enzyme responsible for transcription, exists in different forms across various organisms. In prokaryotes, such as bacteria, there is typically a single type of RNA polymerase that recognizes promoters through its sigma factor subunit. The sigma factor binds to specific sequences within the promoter region, positioning the RNA polymerase correctly for initiation. In contrast, eukaryotic cells have multiple types of RNA polymerase (Pol I, Pol II, and Pol III), each recognizing distinct promoters and requiring different sets of transcription factors for activation. For instance, RNA Polymerase II (Pol II), which transcribes protein-coding genes in eukaryotes, relies on a complex assembly of general transcription factors (GTFs) to facilitate promoter recognition. These GTFs include TFIIA, TFIIB, TFIID, TFIIE, TFIIF, and TFIIH, which collectively help in recruiting Pol II to the promoter and unwinding the DNA double helix. The TATA-binding protein (TBP), a subunit of TFIID, is particularly crucial as it binds to the TATA box, a conserved sequence found in many eukaryotic promoters. In addition to these general factors, specific transcription factors known as enhancer-binding proteins or activators also play significant roles. These proteins bind to enhancer regions located upstream or downstream of the promoter and interact with the GTFs and Pol II to enhance or inhibit transcription. The diversity in these enhancer-binding proteins allows for tissue-specific and developmental stage-specific regulation of gene expression, highlighting the complexity and precision of eukaryotic transcriptional control. Furthermore, the architecture of promoters themselves can vary significantly between prokaryotes and eukaryotes. Prokaryotic promoters often contain conserved sequences such as the -10 and -35 elements that are recognized by the sigma factor. In eukaryotes, however, promoters may include a variety of elements such as the TATA box, CAAT box, and GC box, each serving as binding sites for different transcription factors. The differences in promoter recognition and the involvement of various transcription factors underscore the sophisticated mechanisms that have evolved to regulate gene expression. These mechanisms ensure that genes are transcribed at the right time and in the right place, which is essential for maintaining cellular homeostasis and responding to environmental cues. Understanding these differences not only sheds light on the fundamental biology of transcription but also has implications for fields such as biotechnology and medicine, where precise control over gene expression is crucial.