What Is The Electrostatic Series
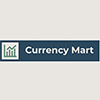
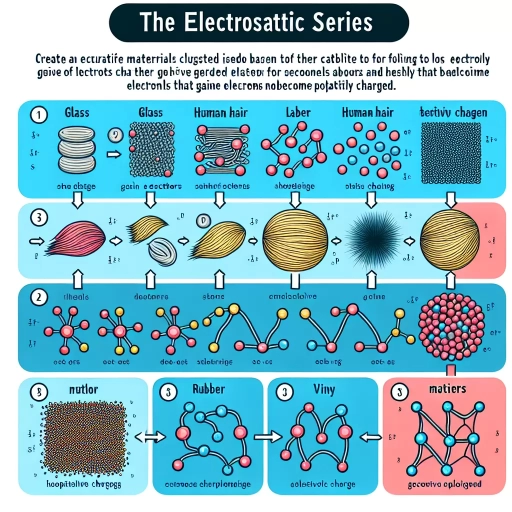
The electrostatic series, a fundamental concept in chemistry and physics, is a sequence of elements arranged according to their ability to attract electrons in a covalent bond. This series is crucial for understanding the chemical properties and reactivity of elements. In this article, we will delve into the intricacies of the electrostatic series, starting with an in-depth explanation of what it is and how it works in the section "Understanding the Electrostatic Series." We will then explore the key elements that define this series, including the factors that influence an element's position within it, in "Key Elements of the Electrostatic Series." Finally, we will examine the practical applications and broader implications of the electrostatic series in various fields, highlighting its significance in "Applications and Implications of the Electrostatic Series." By grasping these aspects, readers will gain a comprehensive understanding of the electrostatic series and its importance in scientific inquiry. Let's begin by understanding the electrostatic series.
Understanding the Electrostatic Series
Understanding the electrostatic series is a fundamental concept in physics and chemistry, offering insights into the behavior of charged particles and their interactions. This article delves into three critical aspects: the definition and basic principles of electrostatics, the historical development and key contributors to the field, and the importance of electrostatics in modern physics and chemistry. By exploring these dimensions, readers will gain a comprehensive understanding of how electrostatic forces shape our world. The journey begins with a clear definition and explanation of the basic principles underlying electrostatics, which form the foundation for grasping more complex concepts. This foundational knowledge sets the stage for appreciating the historical milestones and contributions of scientists who have advanced our understanding of electrostatics over centuries. Finally, recognizing the significance of electrostatics in contemporary scientific research and applications underscores its enduring relevance. Let us start by examining the definition and basic principles of electrostatics, which are essential for a thorough comprehension of this vital subject.
Definition and Basic Principles
The electrostatic series, a fundamental concept in physics and chemistry, is defined as the arrangement of elements in order of their ability to attract electrons in a covalent bond. This series is crucial for understanding the reactivity and properties of elements, particularly in the context of chemical bonding. The basic principles underlying the electrostatic series are rooted in the concept of electronegativity, which is a measure of an atom's ability to attract electrons towards itself in a covalent bond. At its core, the electrostatic series reflects the relative electronegativities of elements. Elements with higher electronegativity values tend to pull electrons closer to themselves, while those with lower values allow electrons to be shared more equally or even repel them towards the less electronegative atom. This principle is essential for predicting the polarity of covalent bonds and the overall stability of molecules. The series typically starts with fluorine (F) as the most electronegative element, followed by other halogens like chlorine (Cl) and bromine (Br), then proceeds through oxygen (O), nitrogen (N), and carbon (C), before reaching less electronegative elements such as hydrogen (H) and metals like sodium (Na) and potassium (K). This ordering helps chemists understand why certain compounds form and how they behave under different conditions. For instance, when fluorine forms a bond with hydrogen, the high electronegativity of fluorine causes the shared electrons to be drawn closer to the fluorine atom, resulting in a polar covalent bond. This polarity can significantly influence the physical and chemical properties of the compound, such as its boiling point, solubility, and reactivity. Understanding the electrostatic series also aids in predicting the types of chemical reactions that can occur. For example, highly electronegative atoms are more likely to participate in ionic bonding due to their strong electron-attracting abilities, while less electronegative atoms may form covalent bonds where electrons are shared more equally. In summary, the electrostatic series is a powerful tool for chemists and physicists, providing a systematic way to understand how elements interact at the atomic level. By recognizing the relative electronegativities of elements and their positions in the series, scientists can better comprehend chemical bonding, predict molecular properties, and explain a wide range of chemical phenomena. This foundational knowledge is essential for advancing our understanding of chemistry and developing new materials and technologies.
Historical Development and Key Contributors
The historical development of the electrostatic series, a fundamental concept in understanding the behavior of ions and their interactions with water, is rooted in the contributions of several key scientists over the centuries. The earliest foundations were laid by Benjamin Franklin, who in the 18th century introduced the concept of positive and negative charges, setting the stage for later research. However, it was Michael Faraday in the 19th century who made significant strides by discovering the laws of electrolysis, which established the relationship between electric charge and chemical reactions. Faraday's work introduced terms such as "ion," "anion," and "cation," which are still central to electrochemistry today. In the late 19th and early 20th centuries, Svante Arrhenius further developed these ideas by proposing the theory of electrolytic dissociation, where salts dissolve in water to form ions. This theory was pivotal in explaining how ions interact with water molecules. Around the same time, Wilhelm Ostwald and Jacobus Henricus van 't Hoff independently worked on the theory of electrolyte solutions, contributing to a deeper understanding of ionic behavior. One of the most influential contributors to the electrostatic series was Fajans, who in the early 20th century developed the Fajans' rules. These rules predict how ions interact with water molecules based on their charge and size, providing a framework for understanding why certain ions are more or less hydrated. Another key figure was Linus Pauling, who introduced the concept of electronegativity and developed a scale that measures an atom's ability to attract electrons in a covalent bond. This work had significant implications for understanding ionic interactions. The electrostatic series itself was formalized through the work of various scientists who studied how ions interact with water and other solvents. The series ranks ions based on their ability to interact with water molecules, with smaller, more highly charged ions being more strongly hydrated. This ranking is crucial for predicting the behavior of ions in solution and has applications in fields ranging from chemistry and biology to engineering and environmental science. In summary, the historical development of the electrostatic series is a testament to the cumulative efforts of numerous scientists over several centuries. From Franklin's initial charge concepts to Faraday's laws of electrolysis, and from Arrhenius' dissociation theory to Fajans' rules and Pauling's electronegativity scale, each contribution has built upon the last to provide a comprehensive understanding of ionic interactions. This rich history underscores the importance of the electrostatic series in modern scientific inquiry and its wide-ranging applications across various disciplines.
Importance in Modern Physics and Chemistry
In modern physics and chemistry, the electrostatic series plays a pivotal role in understanding the fundamental interactions between charged particles. This series, which ranks elements based on their ability to attract electrons, is crucial for predicting chemical reactivity and stability. The electrostatic series helps scientists comprehend how electrons are distributed within atoms and molecules, influencing chemical bonding and reactivity. For instance, in chemistry, the series aids in predicting the formation of ionic bonds by identifying which elements are more likely to lose or gain electrons. In physics, it underpins the study of electrostatic forces that govern the behavior of charged particles at various scales, from atomic interactions to macroscopic phenomena like lightning and static electricity. Understanding the electrostatic series is essential for advancing fields such as materials science, where it guides the development of new materials with specific electrical properties. Additionally, it is vital in biochemistry for elucidating the mechanisms behind enzymatic reactions and protein folding, where electrostatic interactions are key determinants. By grasping the principles of the electrostatic series, researchers can design more efficient batteries, develop new drug molecules, and enhance our understanding of complex biological systems. Thus, the electrostatic series serves as a cornerstone in both physics and chemistry, facilitating deeper insights into the intricate world of charged particles and their interactions.
Key Elements of the Electrostatic Series
The Electrostatic Series is a fundamental concept in chemistry that helps explain the behavior of atoms and molecules. This series is underpinned by several key elements, each contributing to our understanding of chemical properties and reactivity. At the heart of these elements are **Electronegativity and Its Role**, **Ionization Energy and Electron Affinity**, and **Periodic Trends and Patterns**. Electronegativity, for instance, determines how atoms attract electrons in a covalent bond, influencing the polarity and stability of molecules. Ionization energy and electron affinity, on the other hand, reveal the energy required to remove or add electrons to an atom, which is crucial for predicting chemical reactions. Lastly, periodic trends and patterns allow us to systematically analyze and predict these properties across the periodic table. Understanding these interrelated concepts provides a comprehensive framework for analyzing chemical behavior. By delving into these aspects, we can better grasp the underlying principles that govern the electrostatic series. Let's begin by examining **Electronegativity and Its Role**, a critical factor in understanding how atoms interact at the molecular level.
Electronegativity and Its Role
Electronegativity is a fundamental concept in chemistry that plays a crucial role in understanding the electrostatic series. It is a measure of an atom's ability to attract and hold onto electrons in a covalent bond. This property is essential for predicting the distribution of electrons within molecules, which in turn influences various chemical and physical properties. In the context of the electrostatic series, electronegativity helps explain why certain elements tend to form ions with specific charges. For instance, highly electronegative elements like fluorine and oxygen have a strong tendency to attract electrons, often resulting in the formation of anions (negatively charged ions). Conversely, elements with low electronegativity, such as alkali metals like sodium and potassium, readily lose electrons to form cations (positively charged ions). This differential in electronegativity drives the formation of ionic bonds and influences the stability and reactivity of compounds. The electrostatic series, which ranks elements based on their tendency to form ions, is directly influenced by electronegativity. Elements with high electronegativity values are more likely to be found at the top of the series as anions, while those with low electronegativity values are typically at the bottom as cations. Understanding these relationships is vital for predicting chemical behavior and reactivity patterns. Moreover, electronegativity affects the polarity of covalent bonds, which can significantly impact the overall polarity of a molecule. In polar covalent bonds, the difference in electronegativity between the bonded atoms leads to a partial positive charge on one atom and a partial negative charge on the other. This polarity can influence solubility, boiling points, and other physical properties of substances. In summary, electronegativity is a key element in the electrostatic series because it determines how atoms interact with each other in terms of electron attraction and retention. By understanding electronegativity values, chemists can better predict ion formation, bond polarity, and overall chemical behavior, making it an indispensable tool in both theoretical and applied chemistry.
Ionization Energy and Electron Affinity
Ionization energy and electron affinity are crucial elements in understanding the electrostatic series, as they provide insights into the stability and reactivity of atoms. **Ionization energy** is the amount of energy required to remove an electron from a neutral atom in its ground state, typically measured in electronvolts (eV). This energy is a reflection of the atom's ability to hold onto its electrons, with higher ionization energies indicating stronger electron-atom bonds. For instance, noble gases have high ionization energies due to their full outer energy levels, making it difficult to remove an electron. Conversely, alkali metals have low ionization energies because they have a single electron in their outermost shell, which is easily removed. **Electron affinity**, on the other hand, is the energy change when an electron is added to a neutral atom in its ground state. It is also measured in eV and can be either positive or negative, depending on whether energy is released or absorbed during the process. A positive electron affinity indicates that energy is released when an electron is added, suggesting that the atom is more stable with the additional electron. Chlorine, for example, has a high positive electron affinity because adding an electron completes its outer shell, leading to a more stable configuration. In contrast, noble gases generally have negative electron affinities because adding an electron to their full outer shell requires energy. Understanding these concepts is essential for predicting chemical reactivity and stability. For example, elements with low ionization energies and high positive electron affinities tend to be highly reactive because they readily lose and gain electrons to achieve a stable electronic configuration. This reactivity is a key factor in the electrostatic series, where elements are arranged based on their ability to attract electrons and form ions. The interplay between ionization energy and electron affinity helps explain why certain elements are more likely to form cations or anions and how they interact with other elements in chemical reactions. In summary, ionization energy and electron affinity are fundamental properties that influence an atom's chemical behavior. By analyzing these energies, chemists can predict how atoms will react and form compounds, which is central to understanding the electrostatic series and the broader principles of chemistry. These concepts not only help in explaining the periodic trends but also provide a basis for understanding various chemical phenomena, making them indispensable tools in the study of chemistry.
Periodic Trends and Patterns
Periodic trends and patterns are fundamental concepts in chemistry that help predict the properties of elements based on their position in the periodic table. These trends are crucial for understanding how elements behave and interact, which is particularly relevant when discussing the electrostatic series. The electrostatic series, which ranks elements by their ability to attract electrons in a covalent bond, is influenced by several key periodic trends. 1. **Atomic Radius**: As you move from left to right across a period, the atomic radius decreases due to the increasing number of protons in the nucleus, which pulls electrons closer. This decrease in atomic radius results in a higher effective nuclear charge, making elements more electronegative and thus better at attracting electrons. 2. **Electronegativity**: Electronegativity generally increases from left to right across a period and decreases down a group. Elements with higher electronegativity values are more effective at pulling electrons towards themselves, which is a key factor in determining their position in the electrostatic series. 3. **Ionization Energy**: Ionization energy, the energy required to remove an electron from an atom, typically increases across a period and decreases down a group. Elements with high ionization energies are less likely to lose electrons and thus may be less reactive but more electronegative. 4. **Electron Affinity**: Electron affinity, the energy change when an electron is added to an atom, also shows periodic trends. Generally, electron affinity increases across a period but decreases down a group. Elements with high electron affinities are more likely to attract additional electrons, aligning with their position in the electrostatic series. 5. **Shielding Effect**: The shielding effect, where inner electrons shield outer electrons from the full effect of the nuclear charge, decreases down a group. This means that as you move down a group, the effective nuclear charge experienced by outer electrons decreases, leading to lower electronegativity and ionization energy. Understanding these periodic trends allows chemists to predict how elements will behave in various chemical reactions and interactions. For instance, fluorine (F) has the highest electronegativity value due to its small atomic radius and high effective nuclear charge, making it the most effective element at attracting electrons in covalent bonds. Conversely, cesium (Cs) has one of the lowest electronegativities due to its large atomic radius and low effective nuclear charge, making it less capable of attracting electrons. In summary, the electrostatic series is deeply rooted in periodic trends such as atomic radius, electronegativity, ionization energy, electron affinity, and the shielding effect. These trends provide a systematic way to understand why certain elements are more or less effective at attracting electrons in covalent bonds, thereby explaining their positions within the electrostatic series. By grasping these concepts, chemists can better predict chemical behavior and make informed decisions about reactions and compound formation.
Applications and Implications of the Electrostatic Series
The Electrostatic Series, a fundamental concept in physics and chemistry, has far-reaching applications and implications across various scientific disciplines. This series, which describes the electrostatic interactions between charged particles, plays a crucial role in understanding chemical bonding and reactivity, materials science and technology, and biological systems and biochemistry. In the realm of chemical bonding and reactivity, the Electrostatic Series helps explain how ions interact to form compounds and how these interactions influence the stability and properties of molecules. In materials science and technology, it informs the design of materials with specific electrical properties, such as conductors, insulators, and semiconductors. Additionally, in biological systems and biochemistry, electrostatic forces are essential for the structure and function of proteins, DNA, and other biomolecules. Understanding these electrostatic interactions is vital for advancing our knowledge in these fields. This article will delve into these aspects, starting with the critical role of the Electrostatic Series in chemical bonding and reactivity.
Chemical Bonding and Reactivity
Chemical bonding and reactivity are fundamental concepts in chemistry that underpin the understanding of how atoms interact to form molecules and how these molecules participate in chemical reactions. At its core, chemical bonding involves the sharing or transfer of electrons between atoms to achieve a more stable electronic configuration, often mimicking the noble gas configuration. This stability is driven by the electrostatic attraction between positively charged nuclei and negatively charged electrons. The electrostatic series, which ranks elements based on their ability to attract electrons in a covalent bond, plays a crucial role in predicting the reactivity of elements. Elements higher in the electrostatic series, such as fluorine and oxygen, have a strong tendency to attract electrons due to their high electronegativity. This makes them highly reactive as they readily form bonds with other elements to gain electrons and achieve stability. Conversely, elements lower in the series, like cesium and rubidium, are less reactive due to their lower electronegativity and weaker electron-attracting abilities. Understanding these principles has significant implications across various fields. In organic chemistry, knowledge of electrostatic series helps predict the polarity of bonds and the reactivity of functional groups, which is crucial for synthesizing complex molecules. In materials science, it aids in designing materials with specific properties by selecting elements that will form bonds with desired strengths and characteristics. For instance, the high reactivity of fluorine makes it an essential component in fluoropolymers like Teflon, which are known for their non-stick properties and chemical resistance. Moreover, the electrostatic series influences the environmental impact of chemical reactions. For example, the reactivity of certain metals can affect their toxicity and bioavailability in ecosystems. Heavy metals like mercury and lead, which are high in the electrostatic series, tend to form strong bonds with biological molecules, leading to their accumulation and toxicity in living organisms. In pharmaceuticals, understanding the electrostatic series is vital for drug design. The ability to predict how a drug molecule will interact with biological targets, such as proteins and enzymes, relies heavily on the electrostatic properties of the atoms involved. This knowledge helps chemists design drugs that are both effective and safe by minimizing unwanted side reactions. In conclusion, the electrostatic series is a powerful tool for understanding chemical bonding and reactivity. Its applications span from organic synthesis to materials science and environmental chemistry, highlighting the importance of fundamental principles in driving technological advancements and addressing real-world challenges. By leveraging this knowledge, scientists can develop new materials, design more effective drugs, and better manage environmental impacts, underscoring the critical role of electrostatic interactions in shaping our understanding of chemical reactivity.
Materials Science and Technology
Materials Science and Technology play a pivotal role in the development and application of the electrostatic series, a concept that underpins various technological advancements. At its core, materials science is the study of the properties and applications of different materials, such as metals, ceramics, polymers, and composites. This field is crucial for understanding how materials respond to electrostatic charges and how these interactions can be harnessed for practical uses. In the context of the electrostatic series, materials scientists focus on designing and engineering materials that can efficiently generate, store, and manipulate electrostatic charges. For instance, dielectric materials are central to capacitors, which are key components in many electronic devices. The dielectric constant of these materials determines their ability to store electric charge, making them essential for applications ranging from consumer electronics to high-energy storage systems. Moreover, advancements in nanomaterials and nanotechnology have significantly enhanced the performance of electrostatic devices. Nanomaterials with tailored properties can improve the efficiency of electrostatic charging and discharging processes, leading to more powerful and compact devices. For example, graphene and other 2D materials exhibit exceptional electrical conductivity and mechanical strength, making them ideal for next-generation electrostatic applications. The implications of these advancements are far-reaching. In biomedical engineering, electrostatically charged nanoparticles are being explored for targeted drug delivery and tissue engineering. In aerospace, lightweight yet robust materials with enhanced electrostatic properties are critical for developing advanced sensors and communication systems. Additionally, in environmental science, electrostatic precipitators use charged particles to remove pollutants from air streams, contributing to cleaner air quality. From a technological standpoint, the integration of materials science with electrostatic principles has led to the development of innovative products such as electrostatic air purifiers, static eliminators in manufacturing processes, and even self-cleaning surfaces that repel dust and dirt through electrostatic charges. These applications not only improve product performance but also contribute to sustainability by reducing energy consumption and enhancing operational efficiency. In summary, the synergy between materials science and technology is indispensable for the effective application and expansion of the electrostatic series. By continually advancing our understanding of material properties and their interactions with electrostatic charges, we can unlock new possibilities across various industries, driving innovation and improving quality of life. This interdisciplinary approach ensures that the potential of electrostatic technology is fully realized, leading to breakthroughs that transform both everyday life and cutting-edge technological fields.
Biological Systems and Biochemistry
The electrostatic series, a fundamental concept in chemistry, has profound implications and applications in biological systems and biochemistry. At its core, the electrostatic series describes the relative ease with which ions can be reduced or oxidized, influencing various biochemical processes. In biological systems, these electrostatic interactions are crucial for the functioning of proteins, enzymes, and other biomolecules. For instance, the electrostatic properties of amino acids determine the three-dimensional structure of proteins, which in turn affects their catalytic activity and binding affinity. Enzymes, which are biological catalysts, often rely on electrostatic interactions to position substrates correctly for efficient catalysis. Moreover, ion channels and transport proteins utilize electrostatic forces to regulate the flow of ions across cell membranes, maintaining cellular homeostasis and facilitating nerve transmission. In biochemistry, understanding the electrostatic series is essential for predicting redox reactions that occur within cells. These reactions are vital for energy production in mitochondria through the electron transport chain, where electrons are transferred from high-energy molecules to oxygen, generating ATP. The electrostatic series helps biochemists predict which electron carriers will donate or accept electrons, thereby optimizing energy yield. Additionally, it informs the design of drugs that target specific biochemical pathways by modulating redox potentials. For example, certain antioxidants work by neutralizing free radicals through redox reactions, preventing oxidative stress and cellular damage. The implications of the electrostatic series extend to medical diagnostics and therapeutics. In clinical settings, understanding electrostatic interactions aids in the development of biosensors that detect biomarkers for diseases. These sensors often rely on changes in electrostatic properties to signal the presence of specific molecules. Furthermore, knowledge of electrostatic forces guides the engineering of nanoparticles for targeted drug delivery, ensuring that therapeutic agents are delivered efficiently to diseased tissues while minimizing side effects. In summary, the electrostatic series plays a pivotal role in understanding and manipulating biological systems at the molecular level. Its applications span from elucidating protein structure and function to optimizing energy production in cells and designing innovative medical technologies. By grasping these principles, scientists can better comprehend the intricate mechanisms governing life processes and develop more effective treatments for various diseases.