What Is The Payload Of A Rocket
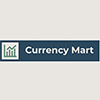
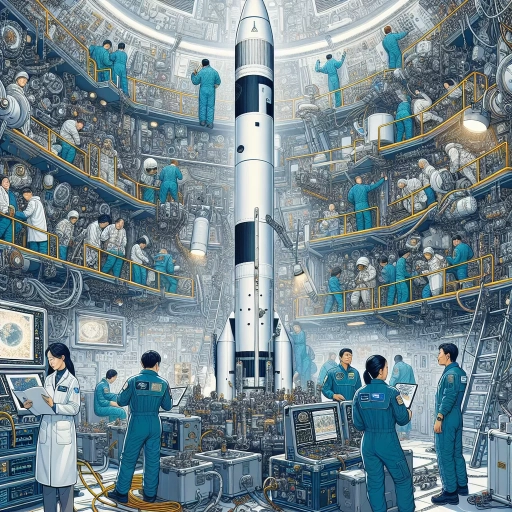
The payload of a rocket is a critical component that defines the primary mission and success of any space launch. Understanding what constitutes the payload is essential for grasping the overall purpose and functionality of a rocket. In this article, we will delve into the intricacies of rocket payloads, starting with a foundational understanding of the concept itself. We will explore the components and structure that make up a rocket payload, highlighting the various elements that contribute to its design and operation. Additionally, we will examine the factors that influence the payload capacity of a rocket, including technological limitations, mission requirements, and environmental considerations. By breaking down these key aspects, readers will gain a comprehensive insight into how payloads are integral to rocket science and space exploration. To begin, let's first understand the concept of payload and its significance in the broader context of rocketry.
Understanding the Concept of Payload
Understanding the concept of payload is crucial for grasping the intricacies of space exploration, technological advancements, and the broader implications of modern engineering. At its core, payload refers to the cargo or equipment carried by a vehicle, such as a rocket, satellite, or aircraft, which is intended to perform a specific function or mission. This article delves into three key aspects that illuminate the significance and evolution of payloads. First, we explore the **Definition and Importance of Payload**, highlighting how payloads are integral to the success of various missions and their impact on scientific research, communication, and economic activities. Next, we examine **Types of Payloads in Rockets**, detailing the diverse range of payloads that have been deployed, from satellites and scientific instruments to crewed spacecraft. Finally, we trace the **Historical Development of Payloads**, chronicling how advancements in technology have shaped the design and functionality of payloads over time. By understanding these facets, we gain a comprehensive insight into the role payloads play in driving innovation and pushing the boundaries of human knowledge. Let us begin by defining and exploring the importance of payloads, as this foundational understanding sets the stage for a deeper appreciation of their types and historical development.
Definition and Importance of Payload
**Definition and Importance of Payload** In the context of rocketry, the payload is the cargo or scientific instruments that a rocket is designed to carry to its intended destination, whether it be into Earth's orbit, to another planet, or beyond. The payload can vary widely depending on the mission objectives, ranging from satellites and space probes to crewed spacecraft and even commercial goods. Understanding the concept of payload is crucial because it directly influences the design, performance, and cost of a rocket. The importance of payload cannot be overstated. For space exploration missions, the payload often includes sophisticated scientific instruments aimed at gathering data about the cosmos. These instruments might include cameras, spectrometers, and sensors that help scientists understand phenomena such as planetary atmospheres, asteroid composition, or cosmic radiation. In commercial applications, payloads can consist of satellites that provide essential services like telecommunications, navigation, and weather forecasting. For instance, communication satellites enable global connectivity by relaying signals across vast distances, while navigation satellites like GPS ensure accurate positioning for various industries including aviation and maritime. Moreover, payloads play a critical role in advancing technological innovation. Space agencies and private companies invest heavily in developing advanced payloads that can withstand the harsh conditions of space travel and operate efficiently once deployed. This drive for innovation has led to significant advancements in materials science, electronics, and software engineering. For example, the development of more efficient solar panels and advanced life support systems for crewed missions has direct implications for improving renewable energy solutions on Earth. The economic importance of payloads is also noteworthy. The launch of commercial satellites generates substantial revenue through subscription services and data sales. Additionally, the data collected by scientific payloads can have far-reaching economic benefits by informing policy decisions related to climate change, natural resource management, and disaster response. For instance, weather satellites provide critical data that helps predict severe weather events, saving lives and reducing economic losses. From a strategic perspective, payloads are integral to national security and defense. Military satellites serve as key assets for surveillance, communication, and navigation, providing critical support for military operations. The ability to deploy and maintain these satellites is a significant factor in a nation's military capability. In summary, the payload is the heart of any rocket mission, driving both the technical and financial aspects of space exploration and utilization. Its importance extends beyond the immediate goals of a specific mission to influence broader societal, economic, and technological advancements. As space technology continues to evolve, understanding the concept of payload remains essential for harnessing the full potential of space travel and exploration.
Types of Payloads in Rockets
When delving into the concept of payloads in rockets, it becomes clear that these components are crucial for the mission's success. A payload is essentially the cargo that a rocket carries to space, and it can vary widely depending on the objectives of the mission. There are several types of payloads, each designed to serve specific purposes. **Scientific Payloads** are among the most common and include instruments such as telescopes, spectrometers, and sensors. These payloads are designed to gather data about space, celestial bodies, or Earth's environment. For instance, NASA's Hubble Space Telescope is a prime example of a scientific payload that has revolutionized our understanding of the universe by capturing high-resolution images and spectra of distant galaxies and stars. **Communication Payloads** are vital for establishing and maintaining communication networks. Satellites equipped with communication payloads provide global connectivity by transmitting data, voice calls, and television signals. These satellites orbit Earth at various altitudes, including geostationary orbits where they remain stationary relative to a fixed point on Earth's surface, ensuring continuous coverage. **Navigation Payloads** play a critical role in providing location information and timing signals. GPS (Global Positioning System) satellites are a prime example of navigation payloads. These satellites transmit signals that allow GPS receivers on Earth to determine precise locations and times, which is essential for navigation in aviation, maritime, and land transportation. **Military Payloads** are used for defense and security purposes. These can include surveillance satellites that monitor enemy movements or communication satellites that support military operations. Some military payloads also involve missile defense systems designed to intercept and destroy incoming threats. **Commercial Payloads** encompass a broad range of applications, including Earth observation satellites that provide imagery for agricultural monitoring, disaster response, and urban planning. Commercial communication satellites also fall under this category, offering services such as broadband internet access and mobile connectivity. **Crewed Payloads** involve spacecraft designed to carry humans into space. These payloads are equipped with life support systems, living quarters, and safety features necessary for sustaining human life during space missions. Examples include the International Space Station (ISS) and crew vehicles like SpaceX's Crew Dragon and NASA's Orion spacecraft. **Experimental Payloads** are used to test new technologies or conduct experimental research in space. These can range from small cubesats (CubeSats) that test new propulsion systems or materials in space to larger experimental platforms like the Bigelow Aerospace's inflatable space habitats. Understanding these different types of payloads highlights the versatility and complexity of rocket missions. Each payload type requires careful design, engineering, and integration to ensure that it meets the specific requirements of its intended mission. By recognizing the diverse roles that payloads play, we gain a deeper appreciation for the intricate planning and execution involved in space exploration and utilization.
Historical Development of Payloads
The historical development of payloads is a fascinating narrative that underscores the evolution of space exploration and technological innovation. The concept of a payload, essentially the cargo or scientific instruments carried by a rocket, has been central to the advancement of space travel since its inception. In the early 1950s, the first payloads were simple in nature, often consisting of small scientific instruments designed to gather basic data about the upper atmosphere and space environment. For instance, the Soviet Union's Sputnik 1, launched in 1957, carried a radio transmitter as its payload, marking the beginning of the space age by providing crucial information about the ionosphere. As rocket technology improved, so did the complexity and sophistication of payloads. The 1960s saw significant milestones with the launch of satellites like Telstar 1, which carried television signals across the Atlantic Ocean, and the Ranger missions to the Moon, which included cameras to capture images of the lunar surface. These early payloads laid the groundwork for more ambitious projects, such as the Apollo missions that successfully landed humans on the Moon. The Apollo command and lunar modules themselves were payloads of the Saturn V rocket, highlighting the critical role these components played in achieving historic feats. The 1970s and 1980s witnessed a proliferation of satellite technology with payloads designed for communication, navigation, and Earth observation. Satellites like Landsat and Seasat were equipped with advanced sensors to monitor Earth's resources and weather patterns. This period also saw the introduction of space stations like Skylab and Salyut, which served as platforms for conducting extensive scientific research in space. These space stations were essentially large payloads that required precise deployment and operation. In recent decades, the diversity and complexity of payloads have expanded exponentially. Modern rockets carry a wide range of payloads, from commercial satellites providing global internet connectivity to sophisticated scientific instruments like those on the Hubble Space Telescope or the Mars Curiosity Rover. The latter, launched in 2011, is a prime example of a highly specialized payload designed to explore Martian geology and search for signs of life. Similarly, the International Space Station (ISS), a collaborative project among multiple space agencies, serves as a large-scale payload that supports ongoing research in microgravity environments. The development of reusable rockets by companies like SpaceX has further revolutionized the field by significantly reducing the cost of launching payloads into space. This innovation has opened up new opportunities for both commercial and scientific missions, enabling more frequent launches and greater flexibility in payload design. For instance, SpaceX's Dragon spacecraft can carry a variety of payloads to the ISS, including crew members, cargo, and specialized scientific equipment. In summary, the historical development of payloads reflects humanity's relentless pursuit of knowledge and technological advancement. From simple radio transmitters to complex scientific instruments and even human beings themselves, payloads have played a pivotal role in shaping our understanding of space and driving innovation in rocket technology. As we continue to push the boundaries of space exploration, the design and deployment of payloads will remain at the forefront of this endeavor.
Components and Structure of a Rocket Payload
The components and structure of a rocket payload are crucial elements that ensure the successful deployment and operation of the payload in space. A comprehensive understanding of these components involves several key aspects, each playing a vital role in the mission's success. First, the **Payload Fairing and Protection Systems** are essential for safeguarding the payload during the ascent phase, protecting it from harsh environmental conditions such as extreme temperatures and aerodynamic forces. Second, **Payload Integration and Interface** systems are critical for ensuring seamless communication and physical compatibility between the payload and the launch vehicle, facilitating smooth operations throughout the mission. Finally, **Payload Deployment Mechanisms** are designed to release the payload into its intended orbit or trajectory, requiring precise engineering to guarantee accurate positioning. Understanding these interconnected components is vital for optimizing payload performance and achieving mission objectives. By delving into these aspects, we can appreciate the complexity and sophistication involved in launching a payload into space. Let's begin by examining the critical role of **Payload Fairing and Protection Systems** in safeguarding the payload during launch.
Payload Fairing and Protection Systems
The payload fairing and protection systems are critical components of a rocket's structure, designed to safeguard the payload during the ascent phase of the launch. The payload fairing, often referred to as the nose cone, is a protective cover that encases the payload and shields it from the harsh conditions encountered during launch, such as extreme temperatures, aerodynamic forces, and debris. Typically made from lightweight yet robust materials like carbon fiber or aluminum, the fairing is jettisoned once the rocket reaches space, where atmospheric pressures are significantly reduced. This jettisoning process is meticulously timed to ensure that the payload is exposed only when it is safe to do so, thereby preventing any potential damage. The protection systems within the payload fairing are equally vital. These include thermal insulation to maintain a stable temperature for sensitive electronics and instruments, as well as vibration dampening mechanisms to mitigate the intense vibrations generated by the rocket's engines. Additionally, these systems often incorporate pressure control mechanisms to maintain a stable internal environment, crucial for payloads that require specific atmospheric conditions. For instance, satellites and spacecraft may need to be kept in a vacuum or at specific pressures to ensure their functionality post-launch. Moreover, the fairing and its associated protection systems must be precisely engineered to accommodate the unique requirements of each payload. This involves detailed structural analysis and testing to ensure that the fairing can withstand the stresses of launch while also providing adequate protection. The integration of these systems with the rocket's guidance and control systems is also essential, allowing for real-time monitoring and adjustments as necessary. In modern rocket design, advancements in materials science and computational modeling have significantly enhanced the performance and reliability of payload fairings. For example, the use of composite materials has reduced weight while increasing strength, allowing for more efficient launches. Similarly, advanced computational fluid dynamics (CFD) and finite element analysis (FEA) enable engineers to optimize fairing designs for minimal drag and maximum structural integrity. The importance of these systems cannot be overstated; any failure in the payload fairing or its protection mechanisms could result in catastrophic consequences for the mission. Therefore, rigorous testing protocols are implemented to validate the performance of these components under various scenarios. This includes ground testing, where the fairing is subjected to simulated launch conditions, as well as flight testing, where actual launch data is collected and analyzed. In summary, the payload fairing and protection systems are indispensable elements of rocket design, ensuring the safe transit of payloads through the Earth's atmosphere into space. Their sophisticated engineering and precise integration with other rocket components underscore their critical role in mission success. As technology continues to evolve, these systems will likely become even more sophisticated, enabling more complex and ambitious space missions in the future.
Payload Integration and Interface
**Payload Integration and Interface** The successful deployment of a rocket payload hinges critically on meticulous payload integration and interface design. This phase involves the precise alignment and connection of the payload with the rocket's structure, ensuring seamless communication and functionality throughout the mission. The integration process begins with a thorough analysis of the payload's specific requirements, including its mass, volume, power consumption, and data transmission needs. Engineers must then design and implement a robust mechanical interface that securely attaches the payload to the rocket's payload fairing or adapter, while also providing necessary thermal protection and vibration damping. A key aspect of this integration is the electrical interface, which facilitates communication between the payload and the rocket's onboard systems. This includes establishing reliable connections for power supply, data transmission, and command reception. The interface must be designed to withstand the harsh conditions of launch, such as extreme vibrations and temperature fluctuations, without compromising signal integrity. Additionally, the integration team must ensure that all interfaces comply with industry standards and mission-specific protocols to avoid compatibility issues. Another crucial element is the development of a comprehensive test plan to validate the integrity of these interfaces. This involves conducting a series of ground tests, including functional checks, environmental tests (such as thermal cycling and vibration tests), and electromagnetic compatibility (EMC) tests. These tests help identify any potential issues early in the integration process, allowing for timely corrections and ensuring that the payload operates as intended once deployed. Furthermore, payload integration often requires sophisticated software tools to manage data exchange and command sequences. These tools enable real-time monitoring and control of the payload during launch and operation, facilitating quick response to any anomalies that may arise. The software must be rigorously tested to ensure it can handle various mission scenarios and contingencies without failure. In addition to technical considerations, logistical aspects such as transportation and handling procedures are also vital. The payload must be transported to the launch site in a manner that preserves its integrity, using specialized containers and handling equipment designed to minimize shock and vibration. At the launch site, careful planning is necessary to ensure that the payload is integrated into the rocket in a timely and efficient manner, adhering strictly to safety protocols. Ultimately, the success of a rocket mission depends on the flawless execution of payload integration and interface design. By combining meticulous planning, rigorous testing, and adherence to stringent standards, engineers can ensure that the payload functions optimally from launch through deployment, delivering valuable data or performing its intended function with precision and reliability. This attention to detail not only enhances mission success but also contributes significantly to advancing our understanding of space and its many mysteries.
Payload Deployment Mechanisms
**Payload Deployment Mechanisms** The successful deployment of a rocket's payload is a critical phase that requires precise and reliable mechanisms. These mechanisms are designed to ensure the payload is released at the correct time, in the correct orientation, and with the necessary velocity to achieve its mission objectives. There are several types of deployment mechanisms, each tailored to specific payload requirements. 1. **Spring-Loaded Mechanisms**: These are among the simplest and most reliable methods. They use stored mechanical energy in springs or other elastic materials to eject the payload from the rocket. Once the rocket reaches the desired altitude or velocity, a pyrotechnic device releases the spring, propelling the payload out of its housing. 2. **Pyrotechnic Separation Systems**: These systems utilize explosive bolts or separation rings to disconnect the payload from the rocket stage. This method is widely used due to its simplicity and high reliability. The pyrotechnic charge is triggered by an onboard computer or a timer, ensuring precise separation. 3. **Pneumatic Deployment**: In this method, compressed gases are used to push the payload out of its container. This approach is often used for smaller payloads where a gentle deployment is necessary to avoid damaging sensitive equipment. 4. **Motorized Deployment**: For more complex missions, motorized systems may be employed. These involve electric motors that slowly and precisely move the payload into its operational position. This method is particularly useful for large or heavy payloads that require careful handling. 5. **Inflatable Structures**: Some payloads, such as solar sails or large antennas, use inflatable structures for deployment. These structures are compactly stored during launch and then inflated once in space, providing a lightweight yet robust solution for expanding large surfaces. 6. **Tethered Deployment**: In some cases, payloads are deployed on a tether attached to the rocket or another spacecraft. This method allows for controlled deployment and retrieval of the payload, which is particularly useful for scientific experiments or satellite servicing missions. Each deployment mechanism must be carefully selected based on the specific needs of the payload, including its size, weight, and operational requirements. The choice of mechanism also depends on factors such as the mission timeline, environmental conditions, and the need for redundancy to ensure mission success. By integrating these sophisticated deployment mechanisms into the rocket's design, engineers can ensure that payloads are delivered accurately and efficiently to their intended destinations in space. This precision is crucial for achieving the scientific, commercial, or military objectives of space missions, making payload deployment mechanisms an indispensable component of rocket technology.
Factors Influencing the Payload Capacity of a Rocket
The payload capacity of a rocket is a critical factor that determines its effectiveness in various space missions. Several key elements influence this capacity, each playing a vital role in the overall performance of the rocket. First, the design and architecture of the rocket significantly impact its payload capacity. The structural integrity, aerodynamics, and weight distribution all contribute to how much payload a rocket can carry efficiently. Second, the propulsion systems and fuel efficiency are crucial as they directly affect the rocket's ability to reach desired altitudes and velocities while carrying a substantial payload. Finally, orbital requirements and mission objectives dictate the specific needs for payload capacity, influencing the design and operational parameters of the rocket. Understanding these factors is essential for optimizing rocket performance and ensuring successful missions. By examining each of these components in detail, we can better appreciate how they interplay to maximize payload capacity. Let's begin by delving into the intricacies of **Rocket Design and Architecture**, which sets the foundation for a rocket's overall capability to carry payloads effectively.
Rocket Design and Architecture
Rocket design and architecture play a crucial role in determining the payload capacity of a rocket, as they directly influence the vehicle's efficiency, stability, and overall performance. The design process involves a meticulous balance of several key factors, including structural integrity, propulsion systems, guidance and control systems, and thermal protection. **Structural Integrity:** The structural framework of a rocket must be robust yet lightweight to maximize payload capacity. Materials such as aluminum alloys, titanium, and advanced composites like carbon fiber are often used due to their high strength-to-weight ratio. The shape and configuration of the rocket's body also impact its aerodynamic characteristics, with streamlined designs reducing drag and enhancing ascent efficiency. **Propulsion Systems:** The choice of propulsion system is critical in rocket design. Liquid-fueled engines offer higher specific impulse (a measure of efficiency) compared to solid-fueled engines but are more complex and heavier. Hybrid engines combine elements of both liquid and solid fuels, offering a balance between simplicity and performance. The number and arrangement of engines also affect payload capacity; for instance, clustered engines can provide greater thrust but may add complexity and weight. **Guidance and Control Systems:** Accurate guidance and control are essential for ensuring that the rocket reaches its intended orbit or trajectory. These systems include navigation software, gyroscopes, accelerometers, and attitude control thrusters. While these components are vital for mission success, they contribute to the overall mass of the rocket, thereby affecting its payload capacity. **Thermal Protection:** During ascent, rockets encounter extreme temperatures due to atmospheric friction. Effective thermal protection systems (TPS) are necessary to shield both the rocket's structure and its payload from these heat loads. Lightweight TPS materials such as ablative coatings or ceramic tiles help maintain structural integrity without significantly increasing the rocket's mass. **Aerodynamics:** Aerodynamic considerations are paramount during the atmospheric phase of flight. The shape of the rocket's nose cone and fairings can significantly reduce drag forces, allowing the vehicle to achieve higher speeds more efficiently. Additionally, features like fins or control surfaces enhance stability during ascent. **Modular Design:** Modern rocket architectures often incorporate modular designs, which allow for easier assembly, testing, and reusability. This modularity can also facilitate the integration of different payloads with minimal adjustments to the rocket's core structure. However, each module adds complexity and potential weight penalties that must be carefully managed. **Reusability:** Reusable rockets have revolutionized the industry by significantly reducing launch costs. Designs that enable recovery and refurbishment of rocket stages after launch can optimize payload capacity over multiple missions. However, the added weight of recovery systems (such as landing legs and grid fins) must be balanced against the benefits of reusability. In summary, the design and architecture of a rocket are intricate processes that involve careful optimization of various components to maximize payload capacity while ensuring mission success. By leveraging advanced materials, efficient propulsion systems, precise guidance and control mechanisms, effective thermal protection, aerodynamic design principles, modular construction techniques, and reusability features, rocket engineers can create vehicles capable of delivering substantial payloads to space efficiently and reliably.
Propulsion Systems and Fuel Efficiency
Propulsion systems and fuel efficiency are crucial factors that significantly influence the payload capacity of a rocket. The primary function of a propulsion system is to generate thrust, which is essential for overcoming the gravitational forces and propelling the rocket into space. There are several types of propulsion systems, each with its own advantages and limitations. For instance, liquid-fueled engines offer high specific impulse (a measure of efficiency) but are complex and require sophisticated management systems. Solid rocket boosters, on the other hand, provide a simpler and more reliable option but with lower specific impulse and less control over thrust. Fuel efficiency plays a pivotal role in determining how much payload a rocket can carry. The specific impulse of an engine, which is a measure of how efficiently it uses propellant to produce thrust, directly affects fuel consumption. Higher specific impulse engines can achieve the same mission requirements with less fuel, thereby allowing for a greater payload capacity. Additionally, advancements in materials and design have led to more efficient combustion chambers and nozzles, further enhancing overall fuel efficiency. Another critical aspect is the type of fuel used. Traditional chemical propulsion systems rely on combinations such as liquid hydrogen and liquid oxygen or kerosene and liquid oxygen. These fuels have well-understood properties but come with their own set of challenges, including storage and handling complexities. Alternative propulsion methods like electric propulsion or advanced ion engines offer even higher specific impulse but require significant power sources, often limiting their application to smaller payloads or longer-duration missions. The design of the rocket itself also impacts fuel efficiency. Aerodynamic considerations during ascent phases can significantly reduce drag, conserving fuel by minimizing energy loss due to atmospheric resistance. Lightweight materials and optimized structural designs further contribute to reducing the overall mass of the rocket, allowing more resources to be allocated towards payload rather than structural integrity. Innovations in propulsion technology continue to push the boundaries of what is possible in terms of payload capacity. Reusability, pioneered by companies like SpaceX, has revolutionized the industry by dramatically reducing launch costs through the recovery and reuse of rocket stages. This not only makes space travel more affordable but also allows for more frequent launches and potentially larger payloads as resources are optimized over multiple missions. In conclusion, the interplay between propulsion systems and fuel efficiency is a cornerstone in determining the payload capacity of a rocket. Advances in engine technology, fuel types, and rocket design all contribute to maximizing the amount of payload that can be carried into space while minimizing the amount of propellant required. As research and development continue to drive innovation in these areas, we can expect even greater efficiencies and capabilities in future rocket missions.
Orbital Requirements and Mission Objectives
When discussing the payload capacity of a rocket, it is crucial to understand the orbital requirements and mission objectives that dictate the design and performance of the launch vehicle. Orbital requirements encompass the specific conditions and parameters necessary for a spacecraft to reach and maintain its intended orbit. These include the desired altitude, inclination, eccentricity, and orbital period. For instance, a satellite intended for geostationary orbit must achieve an altitude of approximately 36,000 kilometers and an inclination of zero degrees relative to the equator. Mission objectives, on the other hand, define the purpose and scope of the mission, such as conducting scientific research, providing telecommunications services, or performing Earth observation. The interplay between these two factors significantly influences the payload capacity of a rocket. For example, a mission aimed at placing a satellite into a high Earth orbit (HEO) or geostationary transfer orbit (GTO) requires more energy than one targeting low Earth orbit (LEO). This increased energy demand translates into a heavier payload fairing, more propellant, and potentially a more powerful rocket, all of which reduce the available payload mass. Conversely, missions with less stringent orbital requirements can often accommodate larger payloads due to the lower energy expenditure. Additionally, mission-specific constraints such as launch window timing, navigation accuracy, and communication requirements further refine the payload capacity. For instance, a planetary mission might necessitate precise trajectory adjustments and robust communication systems, adding to the overall mass budget and potentially reducing the payload capacity. In contrast, a simple LEO deployment might have fewer constraints, allowing for a more generous allocation of mass to the payload. The technological advancements in rocketry also play a critical role in balancing orbital requirements and mission objectives with payload capacity. Modern rockets often employ sophisticated guidance systems, efficient propulsion technologies, and lightweight materials to maximize payload while meeting stringent mission criteria. For example, reusable rockets like those developed by SpaceX have significantly reduced the cost per launch by recovering and reusing major components, thereby enabling larger payloads or more frequent launches. In summary, the payload capacity of a rocket is intricately linked with both the orbital requirements and mission objectives. Understanding these factors allows engineers to optimize rocket design for specific missions, ensuring that the spacecraft reaches its intended orbit while carrying the maximum possible payload. This delicate balance between energy expenditure, technological capabilities, and mission constraints underscores the complexity and sophistication involved in planning and executing space missions. By carefully considering these elements, space agencies and private companies can achieve their mission goals efficiently and effectively.