What Is Mah Battery
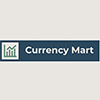
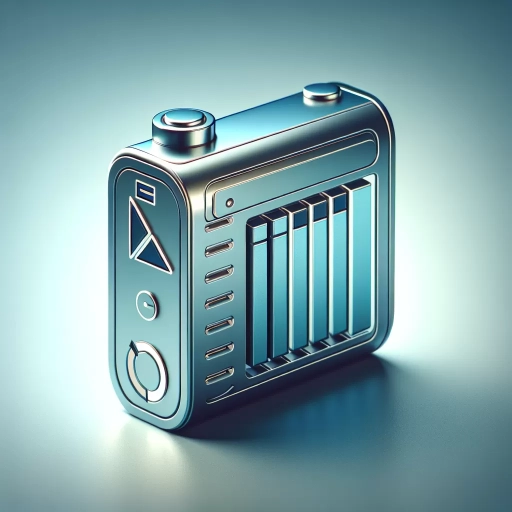
In the modern world, batteries are an indispensable component of our daily lives, powering everything from smartphones and laptops to electric vehicles and renewable energy systems. Understanding what a battery is and how it functions is crucial for appreciating the intricate technology behind these devices. This article delves into the fundamental aspects of battery technology, starting with **Understanding the Basics of Battery Technology**, where we explore the core components and principles that make batteries work. We then dive deeper into **How Batteries Work: The Science Behind the Power**, examining the chemical reactions and physical processes that convert stored energy into usable power. Finally, we look at **Applications and Innovations in Battery Technology**, highlighting the diverse uses of batteries and the cutting-edge advancements that are shaping their future. By grasping these key concepts, readers will gain a comprehensive understanding of the complex yet fascinating world of batteries. Let's begin by understanding the basics of battery technology.
Understanding the Basics of Battery Technology
Understanding the basics of battery technology is crucial for appreciating the intricate mechanisms that power our modern devices. At its core, battery technology involves a complex interplay of chemical reactions, diverse types of batteries, and key components that work in harmony. The chemical composition and reactions within a battery are fundamental to its operation, as they determine the energy storage and release processes. Additionally, distinguishing between primary and secondary batteries is essential, as each type serves different purposes and has unique characteristics. Lastly, the anode, cathode, and electrolyte are the key components that facilitate these chemical reactions, making them indispensable for battery functionality. By delving into these aspects, we can gain a comprehensive understanding of how batteries work. Let's start by examining the chemical composition and reactions that form the backbone of battery technology.
Chemical Composition and Reactions
Chemical composition and reactions are fundamental to understanding the basics of battery technology. A battery operates through a series of electrochemical reactions that convert chemical energy into electrical energy. The primary components of a battery include the anode (negative electrode), cathode (positive electrode), electrolyte (a chemical substance that facilitates ionic conduction), and separators (which prevent the electrodes from coming into contact and causing a short circuit). In a typical lithium-ion battery, for example, the anode is made of graphite, while the cathode is composed of lithium cobalt oxide. When the battery is charged, lithium ions (Li+) move from the cathode through the electrolyte to the anode. During discharge, these ions migrate back to the cathode, releasing electrons that flow through an external circuit, thus generating electricity. This process involves redox (reduction-oxidation) reactions where lithium ions are intercalated into the electrode materials. The chemical composition of these components significantly affects the performance and lifespan of the battery. For instance, different cathode materials like nickel manganese cobalt oxide (NMC) or lithium iron phosphate (LFP) offer varying trade-offs between energy density, safety, and cost. Similarly, advancements in electrolyte chemistry have led to improvements in battery efficiency and safety by reducing the risk of thermal runaway. Understanding these chemical reactions is crucial for optimizing battery design and enhancing overall battery technology. Factors such as charge/discharge cycles, temperature, and state of charge influence the longevity and efficiency of batteries. By manipulating the chemical composition and optimizing reaction kinetics, manufacturers can develop batteries with higher capacities, faster charging times, and longer lifespans. Moreover, ongoing research focuses on developing new electrode materials and electrolytes that can further enhance battery performance. Solid-state batteries, for example, aim to replace liquid electrolytes with solid materials to improve safety and energy density. These advancements underscore the importance of understanding the intricate chemical processes at play in battery technology, driving innovation towards more efficient and sustainable energy storage solutions.
Types of Batteries: Primary vs. Secondary
When delving into the world of battery technology, it is crucial to understand the fundamental distinction between primary and secondary batteries. This dichotomy is pivotal in grasping the basics of battery functionality and application. **Primary Batteries** are non-rechargeable, meaning they can only be used once. Once their chemical energy is depleted, they are discarded. These batteries are typically less expensive to produce and purchase but have a limited lifespan. Primary batteries are commonly used in devices that do not require frequent power replacement, such as remote controls, smoke detectors, and certain medical devices. The most common types of primary batteries include alkaline batteries (e.g., AAA, AA, D) and zinc-carbon batteries. Their simplicity and reliability make them ideal for applications where battery replacement is not a frequent concern. **Secondary Batteries**, on the other hand, are rechargeable. They can be reused multiple times by replenishing their electrical charge through an external power source. This reusability makes them more cost-effective in the long run and environmentally friendly due to reduced waste. Secondary batteries are widely used in portable electronics like smartphones, laptops, and electric vehicles. The most prevalent types of secondary batteries include nickel-cadmium (Ni-Cd), nickel-metal hydride (NiMH), and lithium-ion (Li-ion) batteries. Lithium-ion batteries, in particular, have become ubiquitous due to their high energy density, long cycle life, and relatively low self-discharge rate. The choice between primary and secondary batteries depends on the specific needs of the device or application. For instance, devices that require high power output over a short period may benefit from primary batteries due to their simplicity and reliability. Conversely, devices that need sustained power over an extended period, such as electric vehicles or smartphones, are better suited with secondary batteries due to their rechargeability and efficiency. Understanding the differences between primary and secondary batteries is essential for optimizing performance, reducing costs, and minimizing environmental impact. This knowledge also helps consumers make informed decisions when selecting batteries for their devices, ensuring they choose the most appropriate type for their needs. In the broader context of battery technology, recognizing these distinctions is a foundational step in appreciating the complexities and advancements in this field.
Key Components: Anode, Cathode, and Electrolyte
In the realm of battery technology, understanding the key components is crucial for grasping how batteries function and why they are essential in modern devices. At the heart of any battery are three primary elements: the anode, cathode, and electrolyte. **Anode:** The anode is the negatively charged electrode where chemical reactions occur during the discharge process. It is typically made from materials with high reactivity, such as graphite in lithium-ion batteries. During discharge, the anode releases electrons, which flow through an external circuit to power devices. This process involves the oxidation of the anode material, meaning it loses electrons. **Cathode:** The cathode is the positively charged electrode that complements the anode in a battery. It is usually made from materials with lower reactivity compared to the anode, such as lithium cobalt oxide in lithium-ion batteries. During discharge, the cathode gains electrons from the external circuit, which then combine with ions that have migrated through the electrolyte. This reduction process allows the battery to generate electrical energy. **Electrolyte:** The electrolyte is a chemical substance that facilitates the flow of electrical charge between the anode and cathode. It can be in the form of a liquid, gel, or solid and is designed to be highly conductive while preventing direct contact between the electrodes to avoid short circuits. In lithium-ion batteries, for example, the electrolyte is typically a lithium salt dissolved in an organic solvent. The electrolyte enables ions to move between the electrodes during charge and discharge cycles, allowing the battery to function efficiently. The interaction between these components is what makes battery technology viable. When a battery is connected to a device, such as a smartphone or laptop, chemical energy stored in the anode and cathode is converted into electrical energy. The electrolyte ensures that this conversion process occurs smoothly by facilitating ion transport. Understanding these components not only helps in appreciating how batteries work but also in recognizing their limitations and potential areas for improvement. For instance, advancements in materials science have led to more efficient and durable batteries by optimizing the properties of anodes, cathodes, and electrolytes. This ongoing research is crucial for developing better battery technologies that can meet the increasing demands of modern electronics and renewable energy systems. By grasping the fundamentals of these key components, one can better appreciate the intricate science behind battery technology and its pivotal role in powering our daily lives.
How Batteries Work: The Science Behind the Power
Batteries are the unsung heroes of modern technology, powering everything from smartphones to electric vehicles. Understanding how they work is crucial for appreciating their significance and potential. At the heart of battery operation lies a complex interplay of scientific principles. This article delves into the intricacies of battery functionality, focusing on three key aspects: the role of electrochemical reactions, charge and discharge cycles, and factors affecting battery performance. By exploring these elements, we gain insight into the mechanisms that enable batteries to store and release energy efficiently. The journey begins with the fundamental process of electrochemical reactions, which are the driving force behind a battery's ability to generate power. This foundational concept sets the stage for understanding the broader dynamics of battery operation, including the cyclical nature of charging and discharging, as well as the various factors that influence overall performance. Let's start by examining **The Role of Electrochemical Reactions**.
The Role of Electrochemical Reactions
Electrochemical reactions are the cornerstone of battery functionality, driving the conversion of chemical energy into electrical energy. At the heart of this process are two primary components: the anode and the cathode, separated by an electrolyte. When a battery is connected to a circuit, an electrochemical reaction initiates at the anode, where oxidation occurs. Here, atoms or molecules lose electrons, which then flow through the external circuit to the cathode. Simultaneously, at the cathode, reduction takes place as these electrons combine with ions from the electrolyte, completing the circuit and generating an electric current. This continuous flow of electrons from anode to cathode is what powers devices such as smartphones, laptops, and electric vehicles. The efficiency and longevity of a battery are heavily influenced by the specifics of these electrochemical reactions. For instance, in lithium-ion batteries—a common type used in portable electronics—the anode is typically made of graphite while the cathode is composed of lithium cobalt oxide. During discharge, lithium ions move from the cathode through the electrolyte to the anode, releasing electrons that travel through the external circuit. This process is reversible during charging, allowing lithium-ion batteries to be recharged multiple times. The chemical stability and reactivity of these materials determine how many charge cycles a battery can endure before its capacity diminishes. Moreover, understanding electrochemical reactions is crucial for optimizing battery performance and safety. Factors such as electrode material composition, electrolyte properties, and operating conditions can significantly impact reaction kinetics and overall battery life. For example, high temperatures can accelerate chemical degradation processes within the battery, reducing its lifespan. Conversely, advancements in materials science have led to the development of more efficient and durable electrode materials that enhance battery performance. In addition to their role in powering devices, electrochemical reactions in batteries also highlight broader scientific principles. The principles of thermodynamics and kinetics govern these reactions, influencing how energy is stored and released. Studying these reactions provides insights into fundamental chemical processes that have applications beyond battery technology, such as in fuel cells and supercapacitors. In conclusion, electrochemical reactions are essential for the functioning of batteries, enabling them to convert chemical energy into electrical energy efficiently. Understanding these reactions is vital for improving battery technology, ensuring safety, and exploring new applications in energy storage and conversion. As research continues to advance in this field, we can expect even more efficient and sustainable battery solutions that will power our increasingly technology-driven world.
Charge and Discharge Cycles Explained
Charge and discharge cycles are fundamental concepts in understanding the lifespan and performance of batteries. A charge cycle is a full discharge followed by a full recharge of a battery. For example, if you use 50% of your battery's capacity and then recharge it, that counts as half a cycle. Completing this process—using the remaining 50% and then recharging again—would complete one full cycle. The number of charge cycles a battery can handle before its capacity degrades significantly varies by type and quality of the battery. Lithium-ion batteries, commonly used in portable electronics and electric vehicles, typically last for around 300 to 500 charge cycles before their capacity drops to about 80% of the original. This degradation occurs because each cycle causes minor wear on the internal components of the battery, such as the electrodes and electrolyte. Over time, this wear accumulates, reducing the battery's ability to hold a charge. Other types of batteries have different cycle lifetimes. Lead-acid batteries, often used in cars, may last for around 200 to 300 cycles, while nickel-based batteries can endure more cycles but are less common due to their toxicity and lower energy density compared to lithium-ion. Understanding charge cycles is crucial for maintaining battery health. Avoiding deep discharges (letting the battery drop below 20% capacity) can help extend the battery's lifespan, as shallow discharges are less stressful on the internal components. Additionally, keeping batteries away from extreme temperatures and avoiding overcharging can also help preserve their longevity. In summary, charge and discharge cycles are key metrics in evaluating battery performance and lifespan. By knowing how many cycles a battery can handle and taking steps to minimize wear, users can maximize the life of their batteries and ensure they continue to perform optimally over time. This knowledge is essential for anyone looking to understand how batteries work and how to maintain them effectively.
Factors Affecting Battery Performance
When it comes to understanding how batteries work, it's crucial to delve into the factors that affect their performance. Battery performance is influenced by a multitude of variables, each playing a significant role in determining the overall efficiency and lifespan of the battery. **Temperature** is one of the most critical factors. Extreme temperatures, whether hot or cold, can significantly impact battery performance. High temperatures can accelerate chemical reactions within the battery, leading to faster degradation and reduced lifespan. Conversely, low temperatures slow down these reactions, resulting in lower discharge rates and reduced capacity. For instance, lithium-ion batteries, commonly used in portable electronics, perform optimally between 20°C to 30°C (68°F to 86°F). **Depth of Discharge (DOD)** is another key factor. DOD refers to the percentage of the battery's capacity that is used before recharging. Deep discharging (i.e., using a large portion of the battery's capacity) can reduce the number of charge cycles a battery can handle. Shallow discharging, on the other hand, prolongs battery life but may not be practical for all applications. **Charge Cycles** also play a vital role. A charge cycle is completed when the battery is discharged from 100% to 0% and then recharged back to 100%. The number of charge cycles a battery can handle before its capacity degrades significantly varies by type. For example, lithium-ion batteries typically last for around 300 to 500 charge cycles before their capacity drops to 80% of the original value. **Aging** is an inevitable factor that affects all batteries over time. Even if a battery is not used, it will still degrade due to internal chemical reactions. This process is accelerated by high temperatures and can be mitigated by proper storage conditions such as cool temperatures and partial charging. **Quality of Manufacturing** is also crucial. Batteries produced with high-quality materials and rigorous manufacturing processes tend to perform better and last longer than those made with lower standards. Factors such as electrode material purity, electrolyte quality, and cell design all contribute to overall performance. **Usage Patterns** can vary widely depending on the application. For instance, batteries used in electric vehicles are subjected to different stressors compared to those in consumer electronics. Frequent rapid charging and discharging can reduce battery life more quickly than steady, moderate use. **Environmental Conditions**, such as humidity and exposure to contaminants, can also impact battery performance. Moisture can lead to corrosion within the battery, while contaminants may interfere with internal chemical reactions. Understanding these factors is essential for optimizing battery performance and extending their lifespan. By controlling temperature, managing depth of discharge, monitoring charge cycles, ensuring proper storage conditions, selecting high-quality batteries, adapting usage patterns, and maintaining clean environmental conditions, users can maximize the efficiency and longevity of their batteries. This knowledge not only enhances the functionality of devices but also contributes to more sustainable energy practices by prolonging the useful life of these critical components.
Applications and Innovations in Battery Technology
The rapid evolution of battery technology has transformed various sectors, driving innovation and efficiency across multiple industries. At the forefront of this revolution are advancements in portable electronics and consumer devices, which have seen significant improvements in battery life and performance. However, the impact of battery technology extends far beyond personal gadgets. Electric vehicles and renewable energy systems are also benefiting from these innovations, enabling more sustainable and reliable energy solutions. Furthermore, ongoing research in battery materials and design is pushing the boundaries of what is possible, promising even greater improvements in the future. As we delve into these developments, it becomes clear that the future of energy is being shaped by these advancements. Let's start by examining how these innovations are revolutionizing portable electronics and consumer devices.
Portable Electronics and Consumer Devices
Portable electronics and consumer devices have revolutionized the way we live, work, and communicate. These devices, ranging from smartphones and laptops to smartwatches and wireless earbuds, rely heavily on advancements in battery technology to ensure they are both powerful and portable. The milliampere-hour (mAh) rating of a battery is a critical metric that determines how long these devices can operate on a single charge. For instance, a smartphone with a higher mAh battery can support extended use without needing frequent recharging, making it more convenient for users on the go. Innovations in battery technology have significantly enhanced the performance of portable electronics. Lithium-ion batteries, which dominate the market, offer high energy density and long cycle life, allowing devices to be smaller and lighter while maintaining robust functionality. Advances in materials science have led to the development of more efficient battery chemistries, such as lithium-polymer batteries, which provide better safety and flexibility in design. These advancements enable the creation of thinner, more compact devices that still deliver substantial power. Consumer demand for longer battery life has driven further innovation. Fast charging technologies, for example, allow devices to recharge quickly, often reaching full capacity in under an hour. This feature is particularly beneficial for devices like smartphones and tablets that are used extensively throughout the day. Additionally, power-saving features and energy-efficient designs within these devices help maximize battery life, ensuring that users get the most out of their portable electronics. The integration of battery management systems (BMS) has also played a crucial role in optimizing battery performance. BMSs monitor and control various aspects of battery operation, including charge levels, temperature, and voltage, to prevent overcharging or undercharging and extend the overall lifespan of the battery. This technology is essential for ensuring the reliability and safety of portable electronics. Moreover, the trend towards wireless and wearable technology has pushed the boundaries of battery innovation even further. Devices like smartwatches and fitness trackers require batteries that are not only small but also highly efficient to support continuous operation over several days or even weeks. Here, advancements in low-power consumption and miniaturization have been key, enabling these devices to be both functional and stylish without compromising on battery life. In conclusion, the evolution of portable electronics and consumer devices is closely tied to advancements in battery technology. Higher mAh ratings, improved battery chemistries, fast charging capabilities, and sophisticated battery management systems all contribute to creating devices that are both powerful and portable. As technology continues to advance, we can expect even more innovative solutions that will further enhance the performance and convenience of our everyday electronic devices.
Electric Vehicles and Renewable Energy Systems
The integration of Electric Vehicles (EVs) and Renewable Energy Systems (RES) represents a pivotal convergence in modern technology, driving significant advancements in sustainability and energy efficiency. Electric vehicles, powered by rechargeable batteries, offer a cleaner alternative to traditional fossil fuel-based transportation. When coupled with renewable energy sources such as solar, wind, and hydroelectric power, EVs can operate with minimal environmental impact. This synergy is crucial for reducing greenhouse gas emissions and mitigating climate change. Renewable energy systems provide the necessary infrastructure for charging EVs sustainably. Solar panels, for instance, can be installed at charging stations or even integrated into vehicle designs to generate electricity on the go. Wind turbines and hydroelectric plants can supply power grids that support widespread EV adoption. The use of smart grids and advanced energy storage solutions ensures efficient distribution and storage of renewable energy, making it possible to charge vehicles during periods of low demand or when renewable energy output is high. Innovations in battery technology are central to this ecosystem. Advances in lithium-ion batteries have improved their capacity, lifespan, and charging speed, making EVs more practical for daily use. Solid-state batteries, currently under development, promise even greater improvements in safety, efficiency, and range. These technological strides enable EVs to travel longer distances without recharging and reduce the overall cost of ownership. Moreover, the integration of EVs with renewable energy fosters a more resilient and decentralized energy system. Vehicle-to-Grid (V2G) technology allows EVs to act as energy storage devices, feeding electricity back into the grid during peak demand periods or when renewable energy output is low. This capability helps stabilize the grid and ensures a more reliable supply of clean energy. The economic benefits of this integration are also noteworthy. As the cost of renewable energy continues to decline, charging EVs becomes increasingly cost-effective compared to traditional gasoline-powered vehicles. Additionally, governments worldwide are implementing incentives for both EV adoption and renewable energy investments, further accelerating the transition towards a sustainable transportation sector. In conclusion, the convergence of electric vehicles and renewable energy systems is a transformative force in modern technology. By leveraging innovations in battery technology and integrating these systems with sustainable energy sources, we can significantly reduce our reliance on fossil fuels, lower emissions, and create a more sustainable future for transportation and energy production. This synergy underscores the critical role that advanced battery technologies play in enabling widespread adoption of EVs and fostering a cleaner, more efficient global energy landscape.
Advancements in Battery Materials and Design
Advancements in battery materials and design have revolutionized the field of battery technology, significantly enhancing performance, efficiency, and sustainability. One of the most notable developments is the transition from traditional lead-acid batteries to more advanced chemistries such as lithium-ion (Li-ion) and lithium-iron phosphate (LiFePO4). Li-ion batteries, in particular, have become ubiquitous due to their high energy density, long cycle life, and relatively low self-discharge rate. Innovations in electrode materials, such as the use of graphene and nanomaterials, have further improved the conductivity and surface area of electrodes, leading to faster charging times and higher capacity. The introduction of solid-state electrolytes is another significant advancement. These electrolytes replace the liquid or gel-like substances found in conventional Li-ion batteries, offering enhanced safety by reducing the risk of thermal runaway and explosions. Solid-state batteries also promise higher energy density and faster charging speeds, making them ideal for future electric vehicles and consumer electronics. Design improvements have also played a crucial role. The development of new battery architectures, such as pouch cells and cylindrical cells with optimized internal structures, has allowed for better thermal management and more efficient use of space. Additionally, advancements in battery management systems (BMS) have enabled more precise control over charging and discharging cycles, extending battery lifespan and ensuring optimal performance. Sustainable battery materials are also gaining traction. Researchers are exploring alternatives to cobalt, a key component in many Li-ion batteries but also a source of ethical and environmental concerns. Nickel-rich cathodes and cobalt-free chemistries are being developed to reduce the environmental footprint of battery production. Furthermore, recycling technologies are being developed to recover valuable metals from spent batteries, reducing waste and the demand on primary resources. Innovations in 3D printing and additive manufacturing are also transforming battery design. These techniques allow for the creation of complex geometries that can improve battery performance by increasing surface area and optimizing internal structures. This flexibility in design can lead to batteries that are tailored to specific applications, whether it be for wearable devices, electric vehicles, or renewable energy systems. Overall, these advancements in battery materials and design are driving the evolution of battery technology, enabling more efficient, sustainable, and powerful energy storage solutions that are crucial for a wide range of applications from consumer electronics to electric vehicles and renewable energy systems.