What Are The Building Blocks Of Proteins
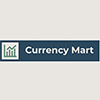
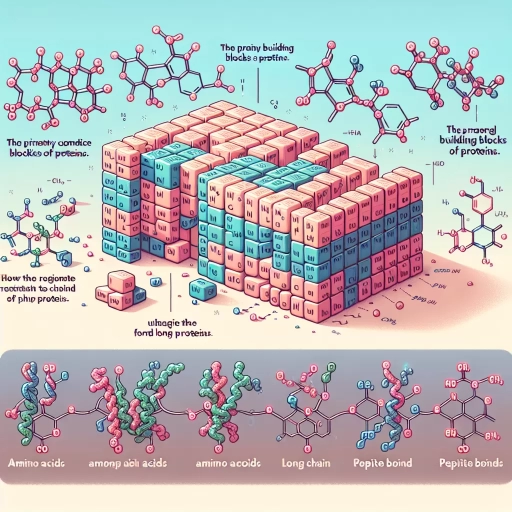
Proteins are the fundamental building blocks of life, playing crucial roles in nearly every biological process. At the heart of their structure and function are amino acids, peptide bonds, and the intricate architecture that arises from their combination. This article delves into the essential components that make up proteins, starting with the foundational role of amino acids. We will explore how these amino acids serve as the primary units of protein construction, followed by an examination of the peptide bonds that link them together. Finally, we will discuss how these elements contribute to the complex structure and diverse functions of proteins. By understanding these building blocks, we gain insight into the molecular machinery that underpins life itself. Let us begin by Understanding Amino Acids, the first and most fundamental component in this intricate biological puzzle.
Understanding Amino Acids
Amino acids are the fundamental building blocks of proteins, playing a crucial role in various biological processes. To fully understand their significance, it is essential to delve into several key aspects. First, the classification of amino acids helps us categorize them based on their chemical properties and side chains, which is vital for understanding their functions and interactions within biological systems. Second, examining the structure and properties of amino acids reveals how their unique configurations influence their reactivity and biological roles. Lastly, distinguishing between essential and non-essential amino acids highlights the importance of dietary intake versus endogenous synthesis. By exploring these dimensions, we can gain a comprehensive understanding of amino acids. Let's begin by examining the classification of amino acids, which serves as a foundational framework for appreciating their diverse roles and characteristics.
Classification of Amino Acids
Amino acids, the fundamental building blocks of proteins, are classified based on their chemical properties and side chain characteristics. There are 20 standard amino acids that the human body uses to construct proteins, each with a unique structure and function. Here’s a concise overview of their classification: 1. **Essential and Non-Essential Amino Acids**: - **Essential Amino Acids** (EAAs): These nine amino acids—histidine, isoleucine, leucine, lysine, methionine, phenylalanine, threonine, tryptophan, and valine—cannot be synthesized by the human body and must be obtained through diet. - **Non-Essential Amino Acids** (NEAAs): The remaining 11 amino acids can be synthesized by the body from other amino acids and do not need to be part of the diet. 2. **Polar and Non-Polar Amino Acids**: - **Polar Amino Acids**: These have side chains that are hydrophilic (water-loving), allowing them to interact with water. They are further divided into: - **Basic Amino Acids**: Arginine, lysine, and histidine have positively charged side chains. - **Acidic Amino Acids**: Aspartic acid and glutamic acid have negatively charged side chains. - **Neutral Polar Amino Acids**: Serine, threonine, asparagine, glutamine, cysteine, and tyrosine have uncharged but polar side chains. - **Non-Polar Amino Acids**: These have hydrophobic (water-fearing) side chains and do not interact with water. They include: - **Aliphatic Amino Acids**: Glycine, alanine, valine, leucine, and isoleucine have non-polar aliphatic side chains. - **Aromatic Amino Acids**: Phenylalanine, tyrosine, and tryptophan have aromatic rings in their side chains. 3. **Charged and Uncharged Amino Acids**: - **Charged Amino Acids**: These include the basic and acidic amino acids mentioned above. - **Uncharged Amino Acids**: This category includes all non-polar and neutral polar amino acids. 4. **Special Cases**: - **Proline**: It is unique because its side chain forms a cyclic structure with the amino group, making it an imino acid rather than a traditional amino acid. - **Glycine**: It is the simplest amino acid with a single hydrogen atom as its side chain, making it non-chiral. Understanding these classifications is crucial for grasping how amino acids contribute to protein structure and function. The diversity in their chemical properties allows proteins to perform a wide range of biological roles, from enzymatic activity to structural support. This classification also aids in predicting protein behavior under different conditions and in designing therapeutic interventions that target specific protein functions.
Structure and Properties of Amino Acids
Amino acids are the fundamental building blocks of proteins, and their structure and properties are crucial for understanding their role in biological systems. Each amino acid consists of a central carbon atom (alpha carbon) bonded to an amino group (-NH2), a carboxyl group (-COOH), a hydrogen atom, and a side chain (R group) that varies among different amino acids. This general structure allows amino acids to form peptide bonds with other amino acids, creating polypeptide chains that eventually fold into proteins. The side chain (R group) of an amino acid determines its unique chemical properties, such as charge, polarity, and hydrophobicity. There are 20 standard amino acids, each with distinct R groups that classify them into different categories: nonpolar (hydrophobic), polar (hydrophilic), acidic, basic, and aromatic. Nonpolar amino acids like alanine and valine have hydrophobic side chains that tend to be buried within the protein's core, while polar amino acids like serine and threonine have hydrophilic side chains that are often exposed on the protein's surface. Acidic amino acids such as aspartic acid and glutamic acid have negatively charged side chains at physiological pH, while basic amino acids like lysine and arginine have positively charged side chains. These charged residues play critical roles in protein function, including enzyme catalysis and protein-protein interactions. Aromatic amino acids like phenylalanine, tyrosine, and tryptophan have large, planar side chains that contribute to the stability and folding of proteins through stacking interactions. The properties of amino acids also influence the overall structure of proteins. For instance, proline is an imino acid with a cyclic side chain that restricts flexibility in the polypeptide backbone, often leading to turns or kinks in the protein structure. Glycine, with its small side chain, provides flexibility to the polypeptide chain, allowing for tighter turns and more compact structures. In addition to their individual properties, amino acids can undergo various post-translational modifications that alter their function and interactions. These modifications include phosphorylation, ubiquitination, and glycosylation, which can regulate enzyme activity, signal transduction pathways, and protein stability. Understanding the structure and properties of amino acids is essential for grasping how proteins are synthesized, folded, and function within cells. The diversity in amino acid side chains allows proteins to perform a wide range of biological functions, from catalyzing metabolic reactions to transmitting signals across cell membranes. This foundational knowledge is critical for fields such as biochemistry, molecular biology, and biotechnology, where understanding protein structure and function is paramount for advancing medical treatments and biotechnological innovations.
Essential vs. Non-Essential Amino Acids
Amino acids are the fundamental building blocks of proteins, and they are categorized into two primary groups: essential and non-essential amino acids. **Essential amino acids** are those that the human body cannot synthesize on its own and must be obtained through diet. There are nine essential amino acids: histidine, isoleucine, leucine, lysine, methionine, phenylalanine, threonine, tryptophan, and valine. These amino acids play critical roles in various bodily functions such as protein synthesis, enzyme production, and hormone regulation. For instance, leucine is crucial for muscle growth and recovery, while tryptophan is involved in the production of serotonin, a neurotransmitter that influences mood and sleep. On the other hand, **non-essential amino acids** can be synthesized by the body from other amino acids. There are 11 non-essential amino acids: alanine, arginine, asparagine, aspartic acid, cysteine, glutamic acid, glutamine, glycine, proline, serine, and tyrosine. Although they can be produced internally, it is still important to consume them through diet to ensure optimal health. Non-essential amino acids are vital for numerous physiological processes; for example, glutamine supports immune function and gut health, while tyrosine is a precursor to neurotransmitters like dopamine and norepinephrine. Understanding the distinction between essential and non-essential amino acids is crucial for maintaining a balanced diet and supporting overall health. Adequate intake of essential amino acids is particularly important for individuals who engage in regular physical activity or have specific dietary needs. For instance, athletes often require higher intakes of essential amino acids to support muscle repair and growth. Conversely, non-essential amino acids, while not as critical in terms of dietary intake, still contribute significantly to various metabolic pathways and should not be overlooked. In summary, both essential and non-essential amino acids are vital components of proteins and play indispensable roles in human physiology. Ensuring adequate intake of all amino acids through a well-rounded diet or appropriate supplementation can help maintain optimal health and support various bodily functions. This understanding underscores the importance of amino acids as the foundational elements of proteins, highlighting their role in sustaining life and promoting well-being.
The Role of Peptide Bonds
Peptide bonds are the fundamental links that connect amino acids to form proteins, which are essential for nearly all biological processes. Understanding the role of peptide bonds is crucial for grasping how proteins are constructed and function. This article delves into three key aspects of peptide bonds: their formation, stability, and role in protein synthesis. First, we explore the **Formation of Peptide Bonds**, detailing the chemical reactions and conditions necessary for these bonds to occur. Next, we examine the **Stability and Strength of Peptide Bonds**, highlighting why these bonds are resilient yet dynamic enough to support the diverse functions of proteins. Finally, we discuss **Peptide Bonding in Protein Synthesis**, explaining how these bonds are integral to the assembly of polypeptide chains during translation. By understanding these facets, we gain insight into the intricate mechanisms that underpin life itself. Let us begin by examining the initial step: the formation of peptide bonds.
Formation of Peptide Bonds
The formation of peptide bonds is a crucial process in the synthesis of proteins, which are the fundamental building blocks of life. This process occurs through a series of biochemical reactions known as peptide bond formation or amide bond formation. Here’s a concise overview: 1. **Initiation**: The process begins with the activation of amino acids, which are the primary components of proteins. Each amino acid has an amino group (NH2) and a carboxyl group (COOH). 2. **Condensation Reaction**: When two amino acids are brought together, they undergo a condensation reaction. In this reaction, the amino group of one amino acid reacts with the carboxyl group of another, resulting in the release of a water molecule (H2O). This reaction is catalyzed by enzymes called peptidyl transferases. 3. **Peptide Bond Formation**: The resulting bond between the two amino acids is called a peptide bond. This bond is a covalent amide bond that links the nitrogen atom of one amino acid to the carbon atom of another, forming a peptide chain. 4. **Polypeptide Chain**: As more amino acids are added to the growing chain through successive peptide bond formations, a polypeptide chain is formed. This chain folds into its native conformation to form a protein, which is determined by the sequence of amino acids. 5. **Role in Protein Synthesis**: Peptide bonds are essential for protein synthesis because they provide the structural framework necessary for proteins to perform their diverse biological functions. The specificity and strength of these bonds ensure that proteins maintain their three-dimensional structure and function correctly. 6. **Energy Requirement**: The formation of peptide bonds requires energy, which is typically provided by ATP (adenosine triphosphate) during protein synthesis. This energy input drives the reaction forward, making it thermodynamically favorable. 7. **Cellular Context**: In cells, peptide bond formation occurs during translation, where messenger RNA (mRNA) sequences are translated into polypeptide chains on ribosomes. The ribosome acts as a molecular factory, aligning amino acids and facilitating the formation of peptide bonds. In summary, the formation of peptide bonds is a critical biochemical process that underpins the synthesis of proteins. Through condensation reactions catalyzed by enzymes, amino acids are linked together via covalent amide bonds to form polypeptide chains, which eventually fold into functional proteins. This process is fundamental to life as it enables the creation of diverse proteins that perform a wide range of biological functions essential for cellular and organismal health.
Stability and Strength of Peptide Bonds
Peptide bonds, the backbone of proteins, exhibit remarkable stability and strength, which are crucial for the structural integrity and functional versatility of proteins. These bonds are formed through a dehydration reaction between the carboxyl group of one amino acid and the amino group of another, resulting in the release of a water molecule and the formation of an amide linkage. The stability of peptide bonds is largely due to their partial double bond character, which arises from resonance between the carbonyl group and the amide nitrogen. This resonance stabilizes the bond, making it resistant to hydrolysis under physiological conditions. Additionally, peptide bonds are strengthened by the planar nature of the amide group, which restricts rotation around the bond axis, contributing to the rigidity and stability of the polypeptide chain. This rigidity is essential for maintaining the three-dimensional structure of proteins, which is vital for their biological functions. Furthermore, the stability of peptide bonds allows proteins to withstand various environmental stresses such as changes in pH and temperature without undergoing significant degradation. The strength and stability of peptide bonds also enable proteins to perform a wide range of biological roles, from enzymatic catalysis to structural support, by maintaining their conformational integrity over time. In summary, the stability and strength of peptide bonds are foundational to the role of proteins in biological systems, ensuring that these complex molecules can fulfill their diverse functions with precision and reliability.
Peptide Bonding in Protein Synthesis
Peptide bonding is a crucial process in protein synthesis, serving as the fundamental mechanism by which amino acids are linked together to form polypeptide chains. During this process, two amino acids come together, and their carboxyl group (-COOH) of one amino acid reacts with the amino group (-NH2) of another, resulting in the formation of a peptide bond. This reaction is known as a dehydration synthesis because it involves the removal of a water molecule (H2O) from the reactants. The peptide bond is a covalent bond that links the carbon atom of the carboxyl group to the nitrogen atom of the amino group, creating an amide linkage. This linkage is stable under physiological conditions, allowing proteins to maintain their structure and function. The formation of peptide bonds is catalyzed by ribosomes during translation, where messenger RNA (mRNA) sequences are decoded into specific amino acid sequences. Transfer RNA (tRNA) molecules bring the appropriate amino acids to the ribosome, aligning them according to the genetic code. The ribosome then facilitates the peptide bond formation between the amino acids, elongating the polypeptide chain one residue at a time. This precise and sequential addition of amino acids ensures that proteins are synthesized with the correct primary structure, which is essential for their proper folding and biological activity. The role of peptide bonds extends beyond mere linkage; they contribute significantly to the overall structure and stability of proteins. The planar nature of the peptide bond restricts rotation around the bond, influencing the secondary, tertiary, and quaternary structures of proteins. This restricted rotation helps in forming alpha helices and beta sheets, which are key components of protein secondary structure. Additionally, peptide bonds participate in hydrogen bonding and other non-covalent interactions that stabilize the three-dimensional conformation of proteins. In summary, peptide bonding is the cornerstone of protein synthesis, enabling the assembly of amino acids into polypeptide chains through dehydration synthesis reactions. These bonds are not only essential for linking amino acids but also play a critical role in determining the structural integrity and functional capabilities of proteins. Understanding peptide bonding is fundamental to grasping how proteins are constructed and how they perform their diverse biological functions.
Protein Structure and Function
Proteins are the building blocks of life, and their structure is intricately linked to their function. Understanding the various levels of protein structure—primary, secondary, tertiary, and quaternary—is crucial for grasping how these biomolecules perform their diverse roles in the body. This article delves into the complexities of protein structure and function, exploring three key aspects: the hierarchical organization of protein structures, the factors that influence these structures, and the relationship between structure and function. By examining the primary, secondary, tertiary, and quaternary structures, we gain insight into how amino acid sequences fold into specific three-dimensional shapes. Additionally, we will discuss the environmental and chemical factors that can alter protein conformation, affecting their functionality. Finally, we will explore how the precise arrangement of atoms within a protein determines its biological activity. This comprehensive approach will provide a thorough understanding of how proteins' intricate structures enable their vital functions. Let us begin by examining the foundational levels of protein organization: primary, secondary, tertiary, and quaternary structures.
Primary, Secondary, Tertiary, and Quaternary Structures
Proteins, the fundamental building blocks of life, exhibit a hierarchical structure that is crucial for their function. This structure is categorized into four levels: primary, secondary, tertiary, and quaternary. **Primary Structure** is the sequence of amino acids that make up a protein. It is determined by the genetic code and is unique to each protein. This linear sequence dictates the overall 3D structure and function of the protein. The primary structure is stabilized by peptide bonds between amino acids. **Secondary Structure** involves local arrangements of amino acids, such as alpha helices and beta sheets, which are stabilized by hydrogen bonds between the backbone atoms of the amino acids. These structures contribute to the protein's stability and play a significant role in its overall conformation. **Tertiary Structure** refers to the overall 3D shape of a single protein molecule. It is determined by interactions between amino acids, including hydrogen bonds, ionic bonds, van der Waals forces, and disulfide bridges. The tertiary structure is essential for the protein's biological activity, as it positions functional groups in a specific spatial arrangement that allows for proper interaction with other molecules. **Quaternary Structure**, applicable to proteins composed of multiple polypeptide chains (subunits), describes how these subunits are arranged in space. The interactions between subunits can be stabilized by the same types of bonds that stabilize tertiary structures. Quaternary structure is critical for proteins that function as enzymes, receptors, or transport proteins, where the arrangement of subunits can affect the protein's activity and regulation. Understanding these structural levels is vital because they collectively determine the protein's function. For instance, enzymes require specific 3D shapes to bind substrates effectively, while receptors need precise structures to interact with ligands. Disruptions in any of these structural levels can lead to protein misfolding and diseases such as Alzheimer's, Parkinson's, and cystic fibrosis. Therefore, the intricate hierarchy of protein structure is a cornerstone of molecular biology and biochemistry, underpinning our understanding of how proteins perform their diverse roles in living organisms.
Factors Influencing Protein Structure
Protein structure is intricately influenced by a variety of factors, each playing a crucial role in determining the final three-dimensional conformation of the protein. **Primary Structure**, the sequence of amino acids, is the foundational blueprint that dictates how the protein will fold. This sequence influences the formation of secondary structures such as alpha helices and beta sheets due to hydrogen bonding patterns between amino acid residues. **Hydrogen Bonding** and **Disulfide Bridges** are key stabilizing forces that contribute to the stability of these secondary structures. Hydrogen bonds form between the backbone carbonyl and amide groups, while disulfide bridges link cysteine residues, providing additional structural rigidity. **Tertiary Structure**, the overall 3D shape of a single protein molecule, is shaped by interactions among amino acid side chains. **Van der Waals Forces**, **Ionic Interactions**, and **Hydrophobic Interactions** all contribute to stabilizing this structure. Hydrophobic amino acids tend to cluster together in the protein core, away from water, while hydrophilic residues are often exposed on the surface. **Protein Folding Pathways** are also critical; chaperone proteins can assist in guiding the folding process to prevent misfolding and ensure proper conformation. **Quaternary Structure**, applicable to multi-subunit proteins, involves interactions between different polypeptide chains. These interactions can be stabilized by similar forces as those in tertiary structure but also involve specific binding sites and interfaces between subunits. **Environmental Factors** such as pH, temperature, and ionic strength can significantly impact protein stability and function by altering these interactions. Additionally, **Post-Translational Modifications (PTMs)** can dramatically influence protein structure and function. PTMs such as phosphorylation, glycosylation, and ubiquitination can introduce new functional groups that alter protein-protein interactions or enzymatic activity. These modifications can be reversible or irreversible and are crucial for regulating various cellular processes. In summary, the structure of proteins is a complex interplay of primary sequence, secondary and tertiary interactions, quaternary arrangements in multi-subunit proteins, environmental conditions, and post-translational modifications. Understanding these factors is essential for appreciating how proteins perform their diverse range of functions within living organisms.
Relationship Between Structure and Function
The relationship between structure and function in proteins is a fundamental concept in biochemistry, highlighting the intricate interplay between the physical arrangement of amino acids and the biological roles these molecules perform. Proteins, composed of sequences of amino acids, fold into specific three-dimensional structures that dictate their functions. This structural specificity arises from the unique properties of each amino acid, including their side chains, which influence the overall conformation of the protein. At the primary level, the sequence of amino acids determines the protein's overall architecture. Secondary structures such as alpha helices and beta sheets are formed through hydrogen bonding between backbone atoms, while tertiary structures involve interactions among side chains, including hydrophobic interactions, ionic bonds, and disulfide bridges. These interactions stabilize the native conformation of the protein, which is crucial for its function. For instance, enzymes, which catalyze biochemical reactions, have active sites with precise geometries that allow them to bind substrates and facilitate chemical transformations. The shape and chemical environment of these active sites are determined by the protein's three-dimensional structure. Similarly, transport proteins like hemoglobin have specific binding sites for oxygen and other ligands, enabling them to transport these molecules across cell membranes efficiently. Structural changes, even minor ones, can significantly impact a protein's function. Mutations in the DNA sequence can lead to alterations in the amino acid sequence, potentially disrupting the protein's native conformation and impairing its ability to perform its biological role. This is evident in diseases such as sickle cell anemia, where a single mutation in hemoglobin causes red blood cells to adopt a sickle shape, leading to severe health complications. Moreover, post-translational modifications like phosphorylation or glycosylation can also modulate protein function by altering its structure. These modifications can change the protein's interactions with other molecules or its stability, thereby regulating its activity. In summary, the structure of a protein is inextricably linked to its function. The precise arrangement of amino acids into secondary, tertiary, and sometimes quaternary structures enables proteins to perform a wide range of biological tasks with high specificity and efficiency. Understanding this relationship is crucial for elucidating protein mechanisms and developing therapeutic strategies to treat diseases related to protein dysfunction.