What Is An Internal Force
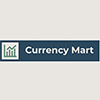
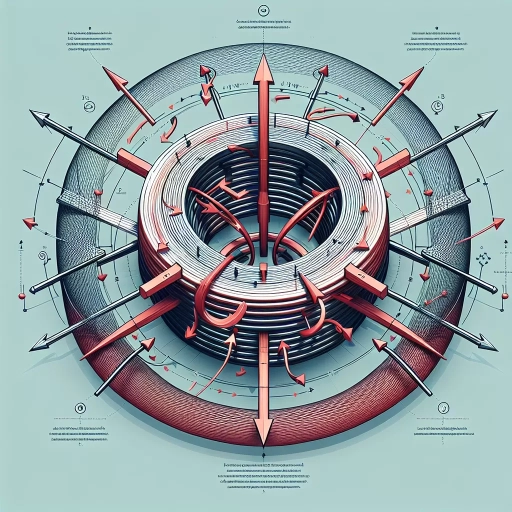
Internal forces are a fundamental concept in physics and engineering, playing a crucial role in understanding the behavior and stability of various structures and systems. These forces arise from within an object or system, influencing its internal dynamics and overall integrity. To delve into the intricacies of internal forces, it is essential to explore their definition and underlying concept, which forms the foundation of our understanding. Additionally, recognizing the diverse types and examples of internal forces helps in applying this knowledge across different fields. Finally, appreciating the impact and importance of these forces highlights their significance in real-world applications. By examining these aspects, we can gain a comprehensive insight into how internal forces shape the world around us. Let us begin by defining and conceptualizing what internal forces are, laying the groundwork for a deeper exploration of their types, examples, and broader implications.
Definition and Concept of Internal Forces
The concept of internal forces is a cornerstone in the fields of physics, engineering, and materials science, offering a profound understanding of how objects behave under various loads. This article delves into the definition and concept of internal forces, providing a comprehensive overview that spans historical, theoretical, and practical dimensions. We begin by exploring the **Historical Context and Development** of internal forces, tracing the evolution of this concept from early scientific inquiries to modern-day applications. This historical perspective sets the stage for a deeper dive into **Scientific Definitions and Theories**, where we examine the fundamental principles and mathematical frameworks that govern internal forces. Finally, we discuss **Practical Applications in Various Fields**, highlighting how these forces are crucial in designing structures, analyzing material properties, and ensuring safety in engineering projects. By understanding the historical roots, scientific underpinnings, and practical uses of internal forces, readers will gain a holistic appreciation for this critical aspect of physical science. Let us start our journey through the historical context and development of internal forces, which have shaped our current understanding and applications.
Historical Context and Development
The concept of internal forces has a rich historical context that spans centuries, evolving from early philosophical and scientific inquiries to the sophisticated understanding we have today. The journey begins with ancient Greek philosophers such as Aristotle, who discussed the nature of motion and the forces that govern it. However, it was not until the work of Galileo Galilei and Sir Isaac Newton in the 16th and 17th centuries that the modern foundation of mechanics was laid. Newton's laws of motion, particularly the third law which states that every action has an equal and opposite reaction, provided a framework for understanding how forces interact within systems. In the 19th century, the development of structural mechanics by engineers like Augustin-Louis Cauchy and Karl Culmann further refined the concept of internal forces. Cauchy's work on stress and strain introduced the idea that materials can resist external loads through internal distributions of force, while Culmann's graphical methods for analyzing structures helped engineers visualize and calculate these forces more accurately. The late 19th and early 20th centuries saw significant contributions from figures like Henri Poincaré and Stephen Timoshenko, who advanced our understanding of elasticity and the behavior of materials under various types of loading. The 20th century witnessed a surge in computational methods and materials science, which dramatically enhanced our ability to analyze and predict internal forces within complex systems. The advent of finite element analysis (FEA) in the mid-20th century allowed for detailed numerical simulations of stress distributions in structures, revolutionizing fields such as aerospace engineering, civil engineering, and biomechanics. This period also saw advancements in materials science, with the development of new materials like composites and smart materials that exhibit unique properties under different types of loading. Today, the study of internal forces is deeply intertwined with advanced computational techniques, experimental methods, and interdisciplinary research. High-performance computing enables detailed simulations that account for non-linear behaviors and dynamic interactions within complex systems. Experimental techniques such as digital image correlation (DIC) and X-ray computed tomography (CT) provide precise measurements of deformation and stress distributions at microscopic scales. Furthermore, interdisciplinary approaches combining mechanics with fields like biology and nanotechnology continue to expand our understanding of how internal forces shape the behavior of diverse materials and structures. In summary, the historical context and development of the concept of internal forces reflect a continuous evolution from foundational principles to sophisticated analytical and computational tools. This journey has been marked by significant contributions from scientists and engineers across various eras, culminating in the robust and multifaceted understanding we possess today. As research continues to advance, our comprehension of internal forces will remain a cornerstone in engineering, science, and technology, enabling innovations that transform industries and improve our daily lives.
Scientific Definitions and Theories
In the realm of physics, scientific definitions and theories form the bedrock upon which our understanding of the natural world is built. When delving into the concept of internal forces, it is crucial to grasp these foundational elements. **Internal forces** are those that act within a system, influencing its behavior and structure. To define and conceptualize internal forces accurately, one must first understand the broader framework of mechanics. **Newton's Laws of Motion**, for instance, provide a cornerstone for analyzing forces. The first law (inertia) states that an object will remain in its state unless acted upon by an external force. However, when considering internal forces, we focus on interactions within the system itself. The second law (force and acceleration) is particularly relevant here, as it relates the net force acting on an object to its mass and acceleration. This law helps in quantifying the effects of internal forces on the system's dynamics. Another key concept is **stress and strain**, which are fundamental in understanding how materials respond to internal forces. Stress refers to the internal forces distributed over a material's cross-sectional area, while strain describes the resulting deformation. These concepts are pivotal in engineering and materials science, where predicting how structures will behave under various loads is essential. **Elasticity theory** further elucidates how materials respond to internal forces by describing their ability to return to their original shape after deformation. This theory introduces concepts like Young's modulus and Poisson's ratio, which quantify a material's stiffness and its lateral response to longitudinal stress, respectively. These parameters are critical in designing structures that must withstand internal stresses without failing. Moreover, **thermodynamics** plays a role in understanding internal forces, especially in systems where temperature changes are involved. The internal energy of a system includes both kinetic energy (due to motion of particles) and potential energy (due to interactions between particles). Changes in internal energy can lead to forces that act within the system, such as those causing expansion or contraction. In addition, **quantum mechanics** offers insights into the microscopic origins of internal forces. At the atomic and subatomic level, forces arise from interactions between particles such as electrons and nuclei. These interactions govern the chemical bonds that hold molecules together and influence the macroscopic properties of materials. Understanding these scientific definitions and theories is essential for comprehending how internal forces shape the behavior of various systems. Whether it's the structural integrity of a bridge, the elasticity of a rubber band, or the thermal expansion of metals, internal forces are at play. By applying these principles, scientists and engineers can design safer, more efficient, and more resilient systems that withstand the stresses imposed by internal forces. In summary, the concept of internal forces is deeply intertwined with fundamental scientific theories and definitions. By grasping Newton's laws, stress and strain, elasticity theory, thermodynamics, and quantum mechanics, we gain a comprehensive understanding of how internal forces operate within different systems. This knowledge is indispensable for advancing our technological capabilities and ensuring the reliability of structures and materials in various fields.
Practical Applications in Various Fields
Internal forces, which arise from the interactions within a system or object, have numerous practical applications across various fields. In **civil engineering**, understanding internal forces is crucial for designing and constructing buildings, bridges, and other structures. Engineers must calculate the stresses and strains on materials to ensure that structures can withstand external loads such as wind, gravity, and seismic activity. For instance, in the design of a skyscraper, internal forces help determine the optimal placement of beams and columns to distribute weight evenly and prevent collapse. In **mechanical engineering**, internal forces play a key role in the design of machinery and mechanisms. For example, in the development of engines, internal forces are analyzed to optimize the performance and durability of components like pistons and crankshafts. This analysis ensures that these components can handle the stresses generated by combustion and motion without failing. In **aerospace engineering**, internal forces are critical for the design of aircraft and spacecraft. The distribution of internal forces within these vehicles must be carefully managed to maintain structural integrity during flight and extreme environmental conditions such as turbulence or re-entry into the Earth's atmosphere. In **biomechanics**, internal forces are studied to understand how living tissues respond to various loads. This knowledge is essential for developing prosthetic devices that mimic the natural behavior of bones and muscles. For instance, understanding the internal forces within joints helps engineers design more effective knee replacements that can withstand the stresses of daily activities. In **materials science**, researchers study internal forces to develop new materials with enhanced properties. By analyzing how internal forces affect material behavior at the atomic or molecular level, scientists can create materials with improved strength, elasticity, or resistance to fatigue. In **medical imaging**, understanding internal forces is vital for techniques like magnetic resonance imaging (MRI) and ultrasound. These technologies rely on the interaction of internal forces within tissues to produce detailed images of the body's internal structures. Furthermore, in **robotics**, internal forces are used to control and stabilize robotic arms and grippers. By sensing and managing internal forces, robots can perform delicate tasks such as assembly or surgery with precision and reliability. Lastly, in **geophysics**, internal forces are studied to understand tectonic plate movements and seismic activity. Analyzing these forces helps scientists predict earthquakes and volcanic eruptions, which is crucial for public safety and disaster preparedness. In summary, internal forces are a fundamental concept with far-reaching practical applications across multiple disciplines. Understanding these forces enables engineers, scientists, and researchers to design safer structures, develop more efficient machinery, create innovative materials, and advance various technologies that improve our daily lives.
Types and Examples of Internal Forces
Internal forces play a crucial role in understanding the behavior and stability of various systems, from the simplest mechanical structures to complex biological organisms. These forces are essential for maintaining the integrity and functionality of objects and systems, and they can be categorized into several key types. One of the primary types is frictional forces, which arise from the interaction between surfaces in contact. Frictional forces are vital in preventing motion and maintaining stability in many mechanical systems. Another significant type is tension and compression forces, which are fundamental in the structural integrity of materials and objects, determining their ability to withstand external loads. Additionally, gravitational forces within objects influence their overall weight distribution and balance. Understanding these internal forces is crucial for designing, analyzing, and optimizing systems across various fields. By delving into each of these categories, we can gain a deeper insight into how internal forces shape the world around us. Let's begin by exploring frictional forces within systems, which are often the unseen heroes in maintaining stability and preventing unwanted motion.
Frictional Forces Within Systems
Frictional forces are a critical component of internal forces within systems, playing a pivotal role in determining the behavior and stability of various mechanical and physical setups. These forces arise from the interaction between surfaces that are in contact, and they can significantly influence the motion, energy dissipation, and overall performance of a system. There are several types of frictional forces, each with distinct characteristics and applications. **Static Friction** is the force that prevents an object from moving when a force is applied to it. It acts in the opposite direction of the applied force and must be overcome for motion to begin. For instance, when you try to push a heavy box across the floor, static friction must be surmounted before the box starts moving. This type of friction is crucial in maintaining stability; without it, objects would slide or roll unpredictably. **Kinetic Friction**, on the other hand, comes into play once an object is already in motion. It opposes the motion of the object and is generally weaker than static friction. Kinetic friction is responsible for the gradual slowing down of moving objects due to energy loss as heat or sound. A common example is the braking system in vehicles, where kinetic friction between the brake pads and the wheels helps to slow down or stop the vehicle. **Rolling Friction** occurs when an object rolls over a surface, such as a wheel on a road or a ball on the ground. This type of friction is typically much weaker than both static and kinetic friction because it involves less surface contact area. However, it still plays a significant role in determining the efficiency of rolling systems, such as in the design of bearings and gears. In addition to these primary types, there is also **Fluid Friction**, which arises when an object moves through a fluid medium like air or water. This force opposes the motion of the object through the fluid and can be seen in phenomena such as air resistance and water drag. For example, an airplane experiences fluid friction as it moves through the air, which affects its speed and fuel efficiency. Understanding and managing frictional forces are essential in various engineering fields. In mechanical engineering, for instance, friction is a key factor in designing machinery and mechanisms where moving parts interact. Lubricants are often used to reduce friction between surfaces, thereby increasing efficiency and reducing wear and tear on components. In civil engineering, frictional forces are critical in ensuring the stability of structures; for example, the friction between soil particles helps to prevent landslides. Moreover, frictional forces have significant implications in everyday life. They are responsible for the grip of tires on roads, which is vital for safe driving. In sports, athletes rely on friction to maintain traction; for instance, soccer players need good friction between their cleats and the ground to run effectively. In conclusion, frictional forces are integral to the functioning of many systems, influencing motion, energy transfer, and stability. By understanding the different types of friction and how they operate within various contexts, engineers and scientists can design more efficient systems and improve performance across a wide range of applications. Whether it's ensuring the safety of vehicles on the road or optimizing the efficiency of industrial machinery, managing frictional forces is a fundamental aspect of internal forces within systems.
Tension and Compression Forces
Tension and compression forces are fundamental types of internal forces that play crucial roles in the structural integrity and behavior of materials. **Tension forces** occur when a material is stretched or pulled apart, causing it to elongate. This type of force is commonly observed in strings, cables, and beams subjected to bending. For instance, when you pull on both ends of a rubber band, the rubber band experiences tension as it stretches. Similarly, in engineering applications, tension forces are critical in the design of suspension bridges where cables are stretched between towers to support the bridge deck. Understanding tension is essential because it helps engineers determine the maximum load a structure can withstand without failing. On the other hand, **compression forces** act to squeeze or compress a material, reducing its volume. These forces are typically seen in columns, pillars, and beams under certain types of loading. For example, when you stand on a spring, it compresses due to the downward force exerted by your weight. In construction, compression forces are vital in the design of building foundations and walls that bear the weight of the structure above them. The ability of a material to resist compression is a key factor in determining its suitability for various applications. Both tension and compression forces are critical in understanding how materials respond to different types of loading. In many real-world scenarios, these forces coexist and interact within a structure. For instance, in a beam subjected to bending, one side experiences tension while the other side experiences compression. This interplay between tension and compression is what allows structures like bridges and buildings to maintain their shape and support loads without collapsing. Moreover, the distinction between tension and compression is not just theoretical; it has practical implications for material selection and structural design. Materials that are strong in tension but weak in compression (like steel) are often used in applications where they will be subjected primarily to tensile forces. Conversely, materials that are strong in compression but weak in tension (like concrete) are used where compressive forces dominate. In summary, understanding tension and compression forces is essential for designing safe and efficient structures. By recognizing how these internal forces act within materials, engineers can predict how structures will behave under various loads, ensuring that they can withstand the stresses imposed upon them. This knowledge is pivotal in fields ranging from civil engineering to aerospace engineering, where the balance between tension and compression can mean the difference between structural integrity and catastrophic failure.
Gravitational Forces Within Objects
Gravitational forces within objects are a fundamental aspect of understanding internal forces, which shape the behavior and structure of various physical systems. Unlike external gravitational forces that act between separate objects, internal gravitational forces operate within the confines of a single object, influencing its internal dynamics. For instance, in celestial bodies like planets and stars, internal gravity plays a crucial role in maintaining their spherical shape and structural integrity. This force ensures that the mass of the object is evenly distributed and that the object remains cohesive under its own weight. In terrestrial contexts, internal gravitational forces are less pronounced but still significant. For example, in large mountains or asteroids, the gravitational pull of the mass at the center of the object affects the distribution of material throughout its volume. This can lead to phenomena such as isostasy in Earth's crust, where the buoyancy of less dense crustal material balances the weight of overlying rock, maintaining equilibrium. The study of internal gravitational forces is also crucial in understanding the stability and evolution of complex systems like galaxies and galaxy clusters. Here, the collective gravitational pull of numerous stars and other celestial entities within these systems governs their overall structure and motion. This collective force is what holds galaxies together despite their vast scales and the high velocities of their constituent parts. Moreover, internal gravitational forces have practical implications in engineering and materials science. For instance, in designing large structures such as skyscrapers or bridges, engineers must consider the internal stresses generated by gravity acting on the material's mass distribution. This ensures that the structure can withstand its own weight as well as external loads without collapsing. In addition to these macroscopic examples, internal gravitational forces have theoretical implications in fields like cosmology and particle physics. The concept extends to hypothetical scenarios involving exotic matter or energy densities where internal gravity could behave differently than in conventional objects. In summary, gravitational forces within objects are a critical component of internal forces that dictate the internal dynamics and stability of various physical systems across different scales—from celestial bodies to terrestrial structures and even theoretical constructs. Understanding these forces is essential for both scientific inquiry and practical applications, highlighting their importance in a comprehensive discussion of internal forces.
Impact and Importance of Internal Forces
The impact and importance of internal forces are multifaceted, influencing various aspects of engineering, physics, and everyday life. At the core of understanding these forces lies their role in maintaining **Structural Integrity and Stability**, ensuring that buildings, bridges, and other structures can withstand external loads without collapsing. Additionally, internal forces play a crucial role in **Energy Transfer and Conservation**, as they govern how energy is distributed and transformed within systems, from mechanical to thermal energy. In practical terms, these principles are essential for **Real-World Engineering and Design Considerations**, guiding engineers in the design of safe, efficient, and durable structures. By examining these dimensions, we can appreciate the profound impact of internal forces on our built environment and the fundamental laws of physics that govern them. This article will delve into each of these areas, starting with the critical role of internal forces in ensuring **Structural Integrity and Stability**.
Structural Integrity and Stability
Structural integrity and stability are paramount in ensuring the safety and longevity of any engineering structure, whether it be a bridge, a skyscraper, or a simple beam. These concepts are intricately linked with the impact and importance of internal forces, which are the forces that act within the material of a structure. Internal forces, such as tension, compression, shear, and torsion, arise from external loads like weight, wind, and seismic activity. The distribution and magnitude of these internal forces determine how well a structure can resist deformation and failure. When a structure is subjected to external loads, the material responds by developing internal forces that counteract these loads. For instance, in a beam under bending, the upper part of the beam is in compression while the lower part is in tension. This balance of internal forces ensures that the beam maintains its shape and does not collapse. The stability of a structure is also influenced by its ability to resist buckling or lateral movement under load. For example, slender columns must be designed to withstand compressive forces without buckling, which can lead to catastrophic failure. The importance of structural integrity cannot be overstated. It directly impacts public safety and economic viability. Structures that fail due to inadequate design or material defects can result in significant loss of life and property damage. Moreover, maintaining structural integrity over time is crucial for extending the lifespan of infrastructure and buildings, thereby reducing maintenance costs and ensuring continuous functionality. Engineers use various methods to analyze and ensure structural integrity, including finite element analysis (FEA) and experimental testing. FEA allows for detailed simulations of how internal forces distribute within a structure under different loading conditions, enabling precise design optimizations. Experimental testing, on the other hand, provides real-world validation of theoretical models and helps in identifying potential weaknesses that may not be apparent through simulations alone. In addition to analytical tools, material selection plays a critical role in maintaining structural integrity. Materials with high strength-to-weight ratios, such as advanced composites or high-strength steels, are often chosen for their ability to resist internal forces effectively while minimizing weight and cost. Furthermore, innovative construction techniques like prestressing and post-tensioning can enhance the stability of structures by preloading them with internal forces that counteract external loads. In conclusion, understanding and managing internal forces are essential for ensuring the structural integrity and stability of any engineering project. By meticulously analyzing how these forces interact within a structure and employing appropriate design strategies and materials, engineers can create safe, durable, and efficient structures that meet both functional requirements and safety standards. This underscores the critical importance of internal forces in the broader context of structural engineering and highlights why their impact should never be underestimated.
Energy Transfer and Conservation
Energy transfer and conservation are fundamental principles that underpin the dynamics of internal forces within systems. When internal forces act upon an object or a system, they can cause changes in its motion, shape, or energy state. This process is governed by the laws of thermodynamics and the principle of conservation of energy. Essentially, energy cannot be created or destroyed; it can only be transformed from one form to another. For instance, in a mechanical system, kinetic energy (the energy of motion) can be converted into potential energy (stored energy) and vice versa. Consider a simple example: when you stretch a rubber band, the elastic potential energy stored in the band increases due to the internal forces acting within its molecular structure. Upon release, this potential energy is converted back into kinetic energy as the rubber band snaps back into its original shape. This transformation illustrates how internal forces facilitate the transfer of energy between different forms while adhering to the principle of conservation. In more complex systems like engines or living organisms, internal forces play a crucial role in energy conversion processes. In an internal combustion engine, chemical energy stored in fuel is converted into thermal energy through combustion, which is then transformed into mechanical energy that powers the vehicle. Similarly, in biological systems, biochemical reactions driven by internal forces convert chemical energy from food into various forms such as kinetic energy for movement or thermal energy for maintaining body temperature. The importance of understanding these processes lies in their impact on efficiency and performance. In engineering applications, optimizing energy transfer mechanisms can significantly enhance the efficiency of machines and reduce energy losses. For example, improving the design of an engine to minimize frictional losses can lead to better fuel economy and reduced emissions. In biological contexts, understanding how internal forces govern metabolic pathways helps in developing treatments for diseases related to energy metabolism disorders. Moreover, the conservation of energy principle has far-reaching implications beyond immediate practical applications. It underscores the interconnectedness of all physical phenomena and highlights the finite nature of our energy resources. Recognizing that all forms of energy are interconvertible but not infinitely available emphasizes the need for sustainable practices and efficient use of resources. In conclusion, the interplay between internal forces and energy transfer is pivotal in understanding how systems function and evolve over time. By grasping these fundamental principles, we can better design, optimize, and manage various systems across different domains—from mechanical engineering to biological sciences—ensuring that we harness energy efficiently while respecting its conservation. This understanding not only enhances our technological capabilities but also fosters a deeper appreciation for the intricate balance that governs our universe.
Real-World Engineering and Design Considerations
In the realm of real-world engineering, understanding and managing internal forces are paramount for the design and construction of structures that are both safe and efficient. Internal forces, which include tension, compression, shear, and torsion, play a crucial role in determining the structural integrity of any engineered system. Engineers must meticulously consider these forces during the design phase to ensure that the structure can withstand various loads without failing. For instance, in bridge construction, internal forces are critical in designing beams and columns that can support the weight of vehicles and pedestrians while resisting external loads such as wind and seismic activity. Similarly, in aerospace engineering, internal forces are essential for designing aircraft components that can endure the stresses of flight, including turbulence and landing impacts. The importance of internal forces extends beyond structural integrity; it also influences material selection and cost optimization. By accurately calculating internal forces, engineers can specify materials that provide the necessary strength without being overly expensive or wasteful. This balance is particularly important in industries where weight and material costs are significant factors, such as in automotive and aerospace engineering. Moreover, understanding internal forces allows engineers to optimize the design for minimal material usage while maintaining performance standards, which can lead to significant cost savings and environmental benefits. In addition to material considerations, internal forces impact the overall lifespan of a structure. Mismanaged internal forces can lead to premature wear and tear, resulting in frequent repairs or even catastrophic failures. For example, in civil engineering projects like dams or high-rise buildings, miscalculations of internal forces could have disastrous consequences. Therefore, engineers employ advanced computational tools and simulation software to model and analyze these forces under various scenarios before actual construction begins. Furthermore, real-world engineering often involves complex systems where multiple components interact dynamically. Here, internal forces must be considered in a holistic manner to ensure that the entire system functions harmoniously. In mechanical engineering, for instance, the design of gearboxes or transmission systems requires a deep understanding of how internal forces distribute across different parts to achieve smooth operation and longevity. Lastly, regulatory compliance is another critical aspect where internal forces come into play. Building codes and industry standards mandate specific safety margins for internal forces to protect public safety and prevent structural failures. Engineers must adhere to these regulations while also innovating within the constraints they impose. In summary, internal forces are a cornerstone of real-world engineering and design considerations. They dictate structural integrity, influence material selection and cost optimization, impact the lifespan of structures, necessitate holistic system design, and ensure regulatory compliance. By meticulously analyzing and managing these forces, engineers can create safe, efficient, and sustainable solutions that meet the demands of modern society.