What Does Atp Mean
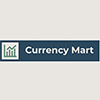
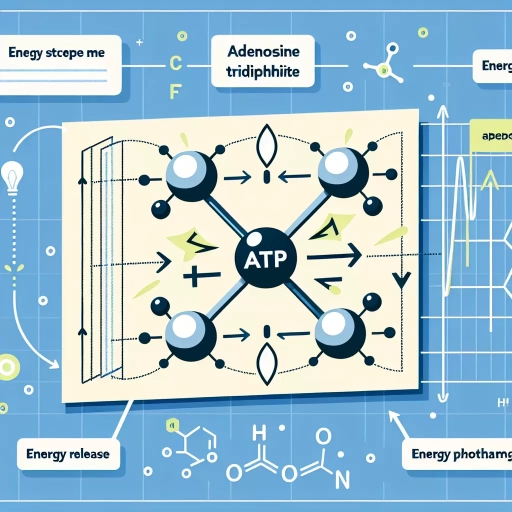
Adenosine triphosphate, commonly abbreviated as ATP, is a molecule that plays a pivotal role in the biological processes of living organisms. It is often referred to as the "molecular currency" of energy transfer within cells. Understanding ATP is crucial for grasping how cells function, how energy is harnessed and utilized, and the intricate mechanisms that sustain life. This article delves into the multifaceted nature of ATP, beginning with **Understanding the Basics of ATP**, where we explore its chemical structure and how it is synthesized and broken down. We then examine **The Biological Functions of ATP**, highlighting its role in energy transfer and its involvement in various cellular activities. Finally, we discuss **ATP in Various Biological Contexts**, illustrating its significance across different biological systems and processes. By the end of this journey, readers will have a comprehensive understanding of ATP's fundamental importance in biology. Let us start by **Understanding the Basics of ATP** to lay the groundwork for this in-depth exploration.
Understanding the Basics of ATP
Adenosine triphosphate, commonly known as ATP, is a molecule that plays a pivotal role in the biological processes of living organisms. Understanding the basics of ATP is crucial for grasping how energy is transferred and utilized within cells. This article delves into three key aspects of ATP: its definition and chemical structure, its role in cellular energy transfer, and the historical discovery and significance of this molecule. By exploring the intricate details of ATP's composition, we gain insight into its unique ability to store and release energy. Additionally, examining its role in cellular energy transfer reveals how ATP acts as a universal energy currency, facilitating various biochemical reactions essential for life. Lastly, tracing the historical discovery of ATP highlights its importance in the field of biochemistry and its impact on our understanding of cellular metabolism. Through these perspectives, we will gain a comprehensive understanding of the basics of ATP, shedding light on its fundamental importance in biological systems. Understanding the basics of ATP is essential for appreciating the intricate mechanisms that sustain life.
Definition and Chemical Structure
**Definition and Chemical Structure** Adenosine triphosphate (ATP) is the molecular currency of energy transfer within cells, playing a pivotal role in various biochemical processes. Defined as a high-energy phosphate compound, ATP is crucial for cellular metabolism, enabling the conversion of chemical energy into mechanical, electrical, and thermal forms. Chemically, ATP consists of three main components: a nitrogenous base called adenine, a sugar molecule known as ribose, and three phosphate groups. The structure of ATP can be visualized as a nucleotide with adenine attached to the 1' carbon of the ribose sugar, which is further linked to a chain of three phosphate groups. The chemical structure of ATP is characterized by its phosphate groups, which are linked through phosphoanhydride bonds. These bonds are high-energy bonds, meaning they require a significant amount of energy to form but release substantial energy when hydrolyzed. This property allows ATP to serve as an efficient energy carrier; when the phosphate groups are cleaved, typically through hydrolysis to form adenosine diphosphate (ADP) and inorganic phosphate, the released energy can be harnessed by the cell to perform various functions such as muscle contraction, protein synthesis, and membrane transport. Understanding the chemical structure of ATP is essential for grasping its functional significance. The phosphate groups are arranged in a linear fashion, with the alpha-phosphate group attached directly to the ribose sugar, followed by the beta-phosphate group, and finally the gamma-phosphate group at the end of the chain. This arrangement facilitates the sequential hydrolysis of phosphate groups, allowing cells to tap into this energy reservoir as needed. Moreover, the unique structure of ATP enables it to interact with a wide range of enzymes and proteins, making it a versatile molecule that can participate in diverse cellular processes. In summary, the definition and chemical structure of ATP underscore its critical role as an energy-rich molecule that powers cellular activities. Its specific arrangement of adenine, ribose, and phosphate groups makes it an ideal energy carrier, capable of releasing and transferring energy efficiently throughout the cell. This fundamental understanding of ATP's structure and function is essential for appreciating its central position in cellular metabolism and energy dynamics.
Role in Cellular Energy Transfer
In the intricate dance of cellular metabolism, ATP (Adenosine Triphosphate) plays a pivotal role in energy transfer, serving as the primary currency of energy within cells. This molecule is crucial for various cellular processes, including muscle contraction, protein synthesis, and membrane transport. The role of ATP in cellular energy transfer can be understood through its unique chemical structure and function. ATP consists of a nucleotide base (adenine), a sugar molecule (ribose), and three phosphate groups. The energy stored in ATP is released when the phosphate bonds are hydrolyzed, converting ATP into ADP (Adenosine Diphosphate) and inorganic phosphate. This process, known as dephosphorylation, liberates a significant amount of energy that can be harnessed by the cell to perform work. The efficiency of ATP in energy transfer lies in its ability to rapidly release and regenerate energy. During cellular respiration, particularly in the mitochondria, ATP is synthesized from ADP and inorganic phosphate using the energy derived from the breakdown of glucose and other organic molecules. This continuous cycle of ATP synthesis and hydrolysis ensures a steady supply of energy for cellular activities. For instance, in muscle cells, the rapid hydrolysis of ATP to ADP provides the necessary energy for muscle contraction, while in nerve cells, ATP fuels the transport of ions across membranes, enabling neural signaling. Moreover, ATP's role extends beyond immediate energy provision; it also regulates various metabolic pathways. For example, ATP can inhibit or activate enzymes involved in glycolysis and the citric acid cycle, thereby modulating the rate of energy production based on cellular needs. This regulatory function ensures that energy production is balanced with energy consumption, maintaining cellular homeostasis. In summary, ATP's central role in cellular energy transfer is multifaceted. It acts as a universal energy carrier, facilitating the conversion of chemical energy into mechanical, electrical, or thermal forms. Its rapid synthesis and hydrolysis cycles make it an efficient and reliable source of energy for diverse cellular processes. Understanding the basics of ATP is essential for grasping how cells manage their energy resources, highlighting the molecule's indispensable role in sustaining life at the cellular level.
Historical Discovery and Significance
The discovery of ATP (Adenosine Triphosphate) is a pivotal moment in the history of biochemistry, underscoring the intricate mechanisms of cellular energy transfer. In the early 20th century, scientists like Karl Lohmann and Fritz Lipmann independently identified ATP as a key energy carrier in cells. This breakthrough was significant because it revealed how cells harness and distribute energy efficiently. ATP's structure, comprising adenine, ribose, and three phosphate groups, was elucidated through meticulous research. The phosphate bonds in ATP are high-energy bonds; when these bonds are hydrolyzed to form ADP (Adenosine Diphosphate) and inorganic phosphate, a substantial amount of energy is released. This energy is crucial for various cellular processes, including muscle contraction, protein synthesis, and membrane transport. The historical significance of ATP's discovery extends beyond its role as an energy currency. It marked a shift in understanding cellular metabolism, highlighting the dynamic interplay between different biochemical pathways. For instance, the citric acid cycle (Krebs cycle) and oxidative phosphorylation were found to generate ATP, while glycolysis and the pentose phosphate pathway contribute to its production in different cellular contexts. This comprehensive view of ATP's role has been instrumental in advancing fields such as molecular biology, genetics, and pharmacology. Moreover, the study of ATP has led to numerous medical and technological advancements. Understanding how ATP is produced and utilized has provided insights into metabolic disorders and diseases, such as mitochondrial myopathies and certain types of cancer. In pharmacology, drugs targeting ATP-dependent processes have been developed to treat conditions like hypertension and heart failure. Additionally, research on ATP has inspired the development of bioenergetic therapies aimed at enhancing cellular energy production. In the broader context of understanding the basics of ATP, its historical discovery serves as a cornerstone. It emphasizes the importance of interdisciplinary research and the continuous pursuit of knowledge in biochemistry. The significance of ATP extends from its fundamental role in cellular metabolism to its implications in medical research and therapeutic applications. As such, appreciating the historical backdrop of ATP's discovery enriches our understanding of its multifaceted importance in biological systems. This foundational knowledge not only deepens our comprehension of cellular processes but also underscores the potential for future scientific breakthroughs in fields reliant on ATP's unique properties.
The Biological Functions of ATP
Adenosine triphosphate (ATP) is the molecular currency of energy within cells, playing a pivotal role in the biological functions that sustain life. This essential molecule is at the heart of cellular metabolism, serving as the primary energy source for various cellular processes. In this article, we will delve into the multifaceted roles of ATP, exploring how it fuels the intricate machinery of cells. We will examine how ATP acts as an **Energy Source for Cellular Processes**, powering everything from muscle contraction to biosynthesis. Additionally, we will discuss the **Mechanism of ATP Synthesis and Breakdown**, highlighting the biochemical pathways that generate and utilize ATP. Finally, we will investigate **Regulation and Homeostasis in Cells**, revealing how cells maintain optimal ATP levels to ensure proper functioning. Understanding these aspects is crucial for grasping the fundamental importance of ATP in biological systems. By the end of this article, you will have a comprehensive understanding of the biological functions of ATP, setting the stage for **Understanding the Basics of ATP**.
Energy Source for Cellular Processes
Energy is the cornerstone of cellular processes, and within cells, this energy is primarily harnessed through the molecule adenosine triphosphate (ATP). ATP serves as the universal energy currency, facilitating a wide array of biological functions that sustain life. The production of ATP is intricately linked to cellular respiration, a metabolic pathway that converts glucose into usable energy. This process begins with glycolysis, where glucose is broken down into pyruvate, generating a small amount of ATP and NADH. The pyruvate then enters the mitochondria, where it undergoes the citric acid cycle (Krebs cycle), producing additional ATP, NADH, and FADH2. These electron carriers (NADH and FADH2) are crucial for the electron transport chain, a series of protein complexes embedded in the mitochondrial inner membrane that generate a proton gradient. This gradient drives the synthesis of ATP through the process of chemiosmosis, significantly amplifying the yield of ATP from each glucose molecule. The efficiency and versatility of ATP make it an indispensable component of cellular metabolism. ATP's high-energy phosphate bonds are easily hydrolyzed to release energy, which is then utilized by various cellular processes such as muscle contraction, protein synthesis, membrane transport, and DNA replication. For instance, during muscle contraction, ATP is hydrolyzed to provide the necessary energy for actin and myosin filaments to slide past each other. Similarly, in protein synthesis, ATP powers the assembly of amino acids into polypeptide chains. The transport of molecules across cell membranes also relies on ATP-driven pumps that maintain ion gradients essential for cellular homeostasis. Moreover, ATP's role extends beyond immediate energy provision; it also acts as a signaling molecule and a cofactor in numerous enzymatic reactions. For example, ATP is involved in the regulation of metabolic pathways through allosteric control of key enzymes. Its presence or absence can signal the cell's energy status, influencing metabolic flux and ensuring that energy-consuming processes are balanced with energy-producing ones. In summary, ATP is the central energy source for cellular processes, generated primarily through cellular respiration and utilized in a myriad of biological functions. Its unique chemical properties make it an ideal energy carrier, allowing cells to efficiently harness and distribute energy as needed. Understanding the biological functions of ATP provides profound insights into the intricate mechanisms that sustain life at the cellular level.
Mechanism of ATP Synthesis and Breakdown
**Mechanism of ATP Synthesis and Breakdown** Adenosine triphosphate (ATP) is the molecular currency of energy in living organisms, and its synthesis and breakdown are crucial for cellular metabolism. The mechanism of ATP synthesis primarily occurs during cellular respiration, a process that converts glucose into energy. This process is divided into three main stages: glycolysis, the citric acid cycle, and oxidative phosphorylation. **Glycolysis** is the initial step where glucose is broken down into pyruvate in the cytoplasm, producing a small amount of ATP and NADH. The **citric acid cycle**, also known as the Krebs cycle or tricarboxylic acid cycle, further breaks down pyruvate into acetyl-CoA, which then enters the mitochondria. Here, it undergoes a series of reactions that produce more ATP, NADH, and FADH2. The majority of ATP synthesis occurs during **oxidative phosphorylation**, which takes place in the mitochondria. In this stage, electrons from NADH and FADH2 are passed through a series of protein complexes in the mitochondrial inner membrane known as the electron transport chain (ETC). As these electrons move down the ETC, they lose energy, which is used to pump protons across the membrane, creating a proton gradient. This gradient drives the enzyme ATP synthase to produce ATP from ADP and inorganic phosphate through a process called chemiosmosis. On the other hand, the breakdown of ATP is a highly regulated process that releases energy for various cellular activities. When cells require energy, ATP is hydrolyzed to ADP and inorganic phosphate, releasing approximately 7.3 kcal/mol of energy. This reaction is catalyzed by enzymes such as ATPases, which are present in various forms and locations within the cell, including the plasma membrane and organelles like the mitochondria. The balance between ATP synthesis and breakdown is tightly regulated to ensure that cells maintain homeostasis and respond to changing energy demands. For instance, during intense muscle activity, ATP is rapidly broken down to fuel muscle contraction, while during periods of rest or low energy demand, ATP synthesis is favored to replenish energy stores. This dynamic interplay underscores the critical role of ATP in supporting the diverse biological functions necessary for life. In summary, the mechanisms of ATP synthesis and breakdown are intricately linked processes that underpin cellular metabolism. Understanding these mechanisms provides insight into how cells manage energy and highlights the central importance of ATP in sustaining life's complex biological functions.
Regulation and Homeostasis in Cells
Regulation and homeostasis in cells are intricate processes that ensure the optimal functioning of cellular activities, and ATP (Adenosine Triphosphate) plays a pivotal role in these mechanisms. Homeostasis, the maintenance of a stable internal environment despite external changes, is crucial for cellular survival. Cells achieve this balance through various regulatory pathways, many of which are energy-dependent and thus rely on ATP. For instance, ion pumps and transport proteins, which maintain the proper ionic balance across cell membranes, require ATP to function. The sodium-potassium pump, a prime example, uses ATP to transport sodium ions out of the cell and potassium ions into the cell, maintaining the necessary electrochemical gradient essential for nerve and muscle function. ATP also fuels the synthesis and degradation of molecules necessary for cellular homeostasis. Enzymes involved in metabolic pathways, such as glycolysis and the citric acid cycle, utilize ATP as a coenzyme or energy source to catalyze reactions that produce or break down vital cellular components. Additionally, ATP is essential for the proper folding and degradation of proteins, processes that are critical for maintaining cellular health and preventing the accumulation of misfolded proteins that can lead to diseases like Alzheimer's and Parkinson's. Furthermore, ATP is integral to signal transduction pathways that regulate various cellular processes. For example, in the phosphoinositide signaling pathway, ATP is used to phosphorylate proteins that activate downstream effectors, leading to responses such as cell growth, differentiation, or apoptosis. This precise regulation ensures that cells respond appropriately to their environment and maintain homeostasis. In addition to these roles, ATP is involved in the regulation of gene expression. Transcription factors, which bind to DNA to initiate or inhibit gene transcription, often require ATP for their activation or translocation into the nucleus. This ensures that genes are expressed at the right time and in the right amount to maintain cellular homeostasis. In summary, ATP is a central molecule in the regulation and homeostasis of cellular functions. Its role in powering ion pumps, metabolic pathways, protein synthesis and degradation, signal transduction, and gene expression underscores its importance in maintaining the delicate balance necessary for cellular health. Without ATP, cells would be unable to regulate their internal environment effectively, leading to dysfunction and potentially death. Thus, understanding the biological functions of ATP provides insight into how cells maintain their intricate balance and why this molecule is so vital for life.
ATP in Various Biological Contexts
Adenosine triphosphate (ATP) is the molecular currency of energy in biological systems, playing a pivotal role in various cellular processes that sustain life. This versatile molecule is essential for muscle contraction and movement, enabling the mechanical work necessary for locomotion, posture, and other physical activities. In the realm of nerve signal transmission, ATP facilitates the rapid communication between neurons, allowing for the swift exchange of information that underpins cognitive functions and sensory perception. Additionally, ATP is a critical component in metabolic pathways and processes, driving the synthesis and breakdown of biomolecules that are vital for cellular homeostasis and energy balance. Understanding the multifaceted roles of ATP in these contexts is crucial for grasping the fundamental mechanisms that govern biological systems. By exploring ATP's functions in muscle contraction, nerve signal transmission, and metabolic pathways, we can delve deeper into the intricate web of cellular processes that rely on this energy-rich molecule. This article will provide an in-depth look at these aspects, ultimately leading to a comprehensive understanding of the basics of ATP.
ATP in Muscle Contraction and Movement
In the intricate dance of muscle contraction and movement, ATP (adenosine triphosphate) plays a pivotal role as the primary energy currency. When a muscle is stimulated to contract, the process begins with the release of calcium ions from the sarcoplasmic reticulum, which then bind to troponin and tropomyosin on the actin filaments. This binding causes a conformational change that exposes myosin binding sites on the actin filaments. Myosin heads, fueled by ATP, undergo a power stroke, pulling the actin filaments towards the center of the sarcomere, thereby shortening the muscle fiber. The energy for this power stroke is derived from the hydrolysis of ATP to ADP (adenosine diphosphate) and inorganic phosphate. This ATP hydrolysis releases a significant amount of energy, which is harnessed to drive the mechanical work of muscle contraction. The efficiency of this process is underscored by the rapid turnover of ATP. During intense muscle activity, ATP stores are depleted within seconds, necessitating rapid replenishment through cellular respiration and anaerobic glycolysis. In aerobic conditions, mitochondria produce ATP through oxidative phosphorylation, utilizing oxygen to generate a high yield of ATP from glucose and fatty acids. In anaerobic conditions, such as during high-intensity, short-duration activities, ATP is replenished through glycolysis and the phosphocreatine kinase reaction, where phosphocreatine donates a phosphate group to ADP to reform ATP. The critical role of ATP in muscle contraction is further highlighted by its involvement in muscle relaxation. After contraction, ATP is required to pump calcium ions back into the sarcoplasmic reticulum and to reposition myosin heads to their resting state, allowing the muscle to relax. This dual function of ATP—both as an energy source for contraction and as a facilitator of relaxation—demonstrates its indispensable role in maintaining muscle function and overall movement. Moreover, the regulation of ATP production and utilization is tightly controlled by various cellular mechanisms to ensure optimal muscle performance. For instance, during prolonged activity, the body adapts by increasing mitochondrial density and enhancing the efficiency of ATP production pathways. This adaptability underscores the dynamic nature of ATP's role in muscle physiology, where it not only fuels immediate contractions but also supports long-term muscle endurance and performance. In summary, ATP is the linchpin of muscle contraction and movement, providing the necessary energy for both the mechanical work of contraction and the regulatory processes of relaxation. Its rapid turnover and replenishment mechanisms ensure that muscles can function efficiently across a range of activities, from brief, high-intensity efforts to sustained, low-intensity tasks. This multifaceted role of ATP in muscle physiology exemplifies its central importance in various biological contexts, where energy demand and supply must be precisely balanced to support life's diverse functions.
ATP in Nerve Signal Transmission
In the intricate process of nerve signal transmission, ATP (adenosine triphosphate) plays a pivotal role, serving as the primary energy currency that fuels the complex biochemical and electrical events involved. When a neuron is stimulated, it generates an action potential—a rapid change in electrical charge across the cell membrane. This process is highly energy-dependent, and ATP is crucial for maintaining the necessary ionic gradients and facilitating neurotransmitter release. At the onset of an action potential, voltage-gated sodium channels open, allowing a rapid influx of sodium ions into the neuron. This influx is followed by the opening of potassium channels, which permit potassium ions to leave the cell, restoring the resting membrane potential. ATP is essential for the operation of sodium-potassium pumps (Na+/K+ ATPases), which actively transport sodium out and potassium into the cell against their concentration gradients, thereby re-establishing the resting potential and preparing the neuron for subsequent action potentials. Moreover, ATP is vital for synaptic transmission—the process by which neurons communicate with each other. When an action potential reaches the presynaptic terminal of a neuron, it triggers the release of neurotransmitters into the synaptic cleft. This release is mediated by exocytosis, a process that involves the fusion of vesicles containing neurotransmitters with the presynaptic membrane. ATP powers this exocytotic machinery through its role in vesicle transport and docking, ensuring that neurotransmitters are efficiently released to bind with receptors on adjacent neurons. Additionally, ATP supports postsynaptic functions by energizing various ion channels and pumps that modulate synaptic strength and plasticity. For instance, ATP-sensitive potassium channels (KATP channels) can modulate neuronal excitability based on metabolic status, providing a link between cellular energy levels and neural activity. In summary, ATP's role in nerve signal transmission is multifaceted and indispensable. It energizes ion pumps to maintain ionic balance, facilitates neurotransmitter release through exocytosis, and modulates synaptic functions to ensure efficient communication between neurons. Without ATP's energy-providing capabilities, the dynamic and precise signaling processes within the nervous system would be severely impaired, highlighting its central importance in maintaining neural function and overall biological integrity.
ATP in Metabolic Pathways and Processes
Adenosine triphosphate (ATP) is the molecular currency of energy in biological systems, playing a pivotal role in various metabolic pathways and processes. Within the context of cellular metabolism, ATP serves as the primary energy carrier, facilitating the transfer of energy from one reaction to another. In glycolysis, for instance, ATP is both consumed and produced; it is used to phosphorylate glucose and fructose-6-phosphate, while later steps in the pathway generate ATP through substrate-level phosphorylation. Similarly, in the citric acid cycle (Krebs cycle), ATP is produced directly through the conversion of succinyl-CoA to succinate. The electron transport chain, a critical component of oxidative phosphorylation, leverages the energy from NADH and FADH2 to pump protons across the mitochondrial membrane, creating a proton gradient that drives ATP synthase to produce ATP. ATP's versatility extends beyond these central metabolic pathways. It is essential for muscle contraction, where it fuels the sliding filament mechanism by binding to myosin heads and enabling their movement along actin filaments. In protein synthesis, ATP powers the assembly of amino acids into polypeptide chains by driving the binding of tRNA to ribosomes and the translocation of ribosomes along mRNA. Additionally, ATP is crucial for membrane transport processes; it fuels the activity of pumps like the sodium-potassium pump, which maintains ion gradients across cell membranes. The significance of ATP in metabolic regulation cannot be overstated. It acts as a key allosteric regulator in many enzymatic reactions, influencing the activity of enzymes involved in glycolysis and gluconeogenesis. For example, high ATP levels inhibit phosphofructokinase-1 and pyruvate kinase in glycolysis, while low ATP levels activate these enzymes to increase energy production. This feedback mechanism ensures that energy production is tightly coupled with energy demand, maintaining cellular homeostasis. Furthermore, ATP's role in signaling pathways underscores its multifaceted nature. It can act as a signaling molecule itself or be involved in the activation of signaling cascades. For instance, extracellular ATP can bind to purinergic receptors on cell surfaces, initiating various physiological responses such as inflammation and neurotransmission. In summary, ATP is indispensable in metabolic pathways and processes, serving not only as an energy carrier but also as a regulatory molecule and signaling agent. Its central role in glycolysis, the citric acid cycle, oxidative phosphorylation, muscle contraction, protein synthesis, membrane transport, and metabolic regulation highlights its importance in maintaining cellular function and overall organismal health. Understanding ATP's multifaceted roles provides insights into the intricate mechanisms that govern life at the cellular level.