What Is Static Load
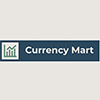
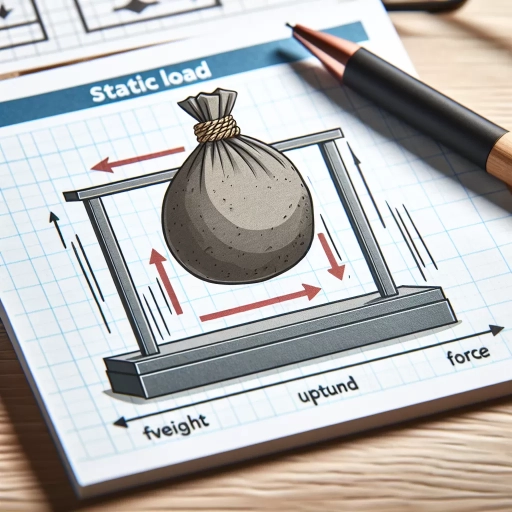
In the realm of engineering and structural analysis, understanding the concept of static load is crucial for ensuring the safety and integrity of buildings, bridges, and other infrastructure. A static load refers to a constant force applied to a structure over a period of time, which can significantly impact its design and construction. This article delves into the intricacies of static loads, beginning with a comprehensive definition and exploration of the basics in "Understanding Static Load: Definition and Basics." Here, we will dissect the fundamental principles that govern static loads and how they differ from dynamic loads. We will then proceed to "Calculating and Analyzing Static Loads," where we will discuss the methodologies and tools used to quantify and assess these forces. Finally, in "Applications and Real-World Examples of Static Loads," we will examine how static loads are applied in various engineering contexts, providing tangible examples that illustrate their practical significance. By grasping these concepts, engineers and architects can better design structures that withstand the stresses of static loads, ensuring durability and reliability. Let us start by understanding the core of what constitutes a static load.
Understanding Static Load: Definition and Basics
Understanding static load is a fundamental concept in engineering and design, crucial for ensuring the structural integrity and safety of various systems and structures. At its core, static load refers to the forces that act on a system without causing it to move or change its position over time. This concept is multifaceted and can be broken down into several key areas. First, it is essential to grasp the **Definition of Static Load**, which involves understanding the types of forces involved and how they are applied. Next, recognizing the **Types of Static Loads**—such as dead loads, live loads, and environmental loads—helps engineers and designers to accurately predict and manage these forces. Finally, appreciating the **Importance in Engineering and Design** highlights how static load calculations are pivotal in preventing failures and ensuring compliance with safety standards. By delving into these aspects, professionals can better navigate the complexities of static load analysis. Let's begin by exploring the **Definition of Static Load** in more detail.
Definition of Static Load
**Understanding Static Load: Definition and Basics** A **static load** is a type of load that remains constant over time and does not change in magnitude or direction. This concept is fundamental in various fields such as engineering, physics, and architecture, where understanding the behavior of structures under different types of loads is crucial. Unlike dynamic loads, which vary over time due to factors like vibrations or impacts, static loads are steady and predictable. For instance, the weight of a building itself or the weight of furniture and occupants in a room are examples of static loads. These loads exert a continuous force on the structure without any fluctuations, allowing engineers to design and analyze structures with greater precision. In engineering design, static loads are often considered first because they provide a baseline for structural integrity. For example, when designing a bridge, engineers must account for the static load of the bridge's own weight as well as the static load of expected traffic. This includes calculating the weight distribution across different parts of the bridge to ensure that it can support these loads without failing. The analysis of static loads involves determining the maximum stress and strain that a structure can withstand under these constant forces. This process typically involves using mathematical models and computational tools to simulate how different materials and structural configurations respond to static loading conditions. The definition of static load also encompasses external forces that act on a structure in a consistent manner. For instance, wind pressure on a building can sometimes be treated as a static load if it is relatively constant over time, although it may more accurately be described as a quasi-static load due to slight variations. Similarly, soil pressure on foundation walls or hydrostatic pressure on dams are other examples where static loads play a significant role in structural design. Understanding static loads is essential for ensuring the safety and durability of structures. Misjudging these loads can lead to catastrophic failures, as seen in historical cases where buildings or bridges have collapsed due to inadequate design or material selection. Therefore, engineers employ rigorous testing and simulation techniques to validate their designs against static loading conditions. This includes conducting material strength tests, finite element analysis, and other forms of structural analysis to guarantee that the structure can withstand the anticipated static loads over its lifespan. In summary, the concept of static load is pivotal in structural engineering and design. It represents a consistent force that structures must endure without failure, making it a critical factor in ensuring the stability and longevity of buildings, bridges, and other infrastructure. By accurately defining and analyzing static loads, engineers can create robust designs that meet safety standards and perform optimally under expected conditions. This foundational understanding sets the stage for more complex analyses involving dynamic loads and other environmental factors that structures may encounter.
Types of Static Loads
Static loads are forces that act on a structure or object without changing over time, and they can be categorized into several types based on their nature and application. **Dead Loads** are the most straightforward type, consisting of the weight of the structure itself, including all permanent components such as walls, floors, roofs, and fixed equipment. These loads are constant and predictable, making them easier to account for in structural design. **Live Loads**, on the other hand, are dynamic in the sense that they can vary in magnitude and location. These loads include the weight of people, furniture, and movable equipment that may be present on a structure at any given time. Live loads are typically estimated based on the intended use of the space; for example, a residential building will have different live load requirements compared to an office building or a warehouse. **Environmental Loads** encompass forces exerted by natural elements such as wind, snow, and earthquakes. **Wind Loads** are particularly significant for tall structures or those located in areas prone to high winds. These loads can cause both horizontal and vertical stresses on a building due to pressure differences around it. **Snow Loads** are critical in regions with heavy snowfall, as they can accumulate on roofs and other surfaces, adding substantial weight that must be supported by the structure. **Earthquake Loads** are another form of environmental load that can impose severe stresses on structures due to ground motion during seismic events. These loads are highly variable and depend on factors such as the seismic zone of the location and the type of soil beneath the foundation. In addition to these primary types, there are also **Impact Loads** which occur when objects collide with a structure. These could be accidental impacts from vehicles or machinery or intentional impacts such as those from construction activities. **Frictional Loads** arise from the resistance between surfaces in contact with each other; while not always significant, they can be crucial in certain applications like bearings and gears. Understanding these different types of static loads is essential for engineers and architects to ensure that structures are designed and constructed to withstand various forces safely and efficiently. By accurately calculating and accounting for these loads during the design phase, professionals can mitigate risks associated with structural failure and ensure compliance with building codes and standards. This comprehensive approach not only enhances safety but also optimizes resource usage by avoiding unnecessary over-engineering or under-engineering of structural elements. In summary, recognizing the diverse nature of static loads—whether they are dead, live, environmental (such as wind or snow), impact-related, or frictional—is fundamental to creating robust and reliable structures that meet both functional needs and safety standards. This nuanced understanding allows for better planning, design execution, and long-term maintenance of buildings and other engineered systems.
Importance in Engineering and Design
In the realm of engineering and design, understanding static load is paramount for ensuring the structural integrity and safety of various systems and structures. Static load, which refers to forces that do not change over time, plays a crucial role in determining the stability and durability of buildings, bridges, machinery, and other engineered constructs. The importance of static load analysis cannot be overstated; it is a fundamental aspect of design that helps engineers predict how a structure will behave under constant forces such as gravity, weight, and environmental pressures. When engineers design a new structure or system, they must meticulously calculate the static loads it will endure. This involves considering the weight of the structure itself, as well as any external loads it may bear, such as the weight of occupants, furniture, or cargo. For instance, in civil engineering, static load calculations are essential for designing foundations that can support the weight of a building without compromising its stability. Similarly, in mechanical engineering, understanding static loads is critical for designing machinery components that can withstand constant stresses without failing. The accuracy of these calculations directly impacts the safety and performance of the final product. Incorrectly assessing static loads can lead to catastrophic failures, resulting in significant economic losses and potential harm to people. For example, a bridge that is inadequately designed to handle static loads may collapse under the weight of traffic, while a machine component that is not engineered to withstand static stresses may fail prematurely, leading to downtime and maintenance costs. Moreover, static load analysis is not just about ensuring safety; it also influences the efficiency and cost-effectiveness of a design. By optimizing the structure to handle static loads effectively, engineers can minimize material usage while maintaining structural integrity. This not only reduces construction costs but also enhances the overall sustainability of the project by reducing waste and environmental impact. In addition to these practical considerations, understanding static load is foundational to more advanced engineering concepts. It serves as a baseline for dynamic load analysis, which involves forces that change over time due to factors like vibration or impact. Without a solid grasp of static loads, engineers would be unable to accurately predict how structures will respond to dynamic forces, further complicating the design process. In conclusion, the importance of understanding static load in engineering and design cannot be overstated. It is a critical component of ensuring structural safety, optimizing design efficiency, and laying the groundwork for more complex analyses. As engineering continues to evolve with new materials and technologies, the fundamental principles of static load analysis remain a cornerstone upon which all successful designs are built. By mastering this concept, engineers can create robust, reliable, and sustainable solutions that meet the demands of modern society while safeguarding public safety.
Calculating and Analyzing Static Loads
Calculating and analyzing static loads is a critical aspect of engineering and structural design, ensuring the safety and integrity of buildings, bridges, and other infrastructure. This process involves several key components that must be meticulously considered to achieve accurate and reliable results. First, understanding the methods for calculating static loads is fundamental, as it lays the groundwork for all subsequent analyses. These methods involve determining the weight and distribution of loads on a structure, which can include dead loads from the structure itself and live loads from occupants or external forces. Additionally, the tools and software used in analysis play a pivotal role in streamlining and enhancing the accuracy of these calculations. Advanced software can simulate various load scenarios, providing valuable insights into how structures will behave under different conditions. However, common challenges and considerations must also be addressed, such as material properties, environmental factors, and potential sources of error. By mastering these elements, engineers can ensure that their designs are robust and resilient. In this article, we will delve into the methods for calculating static loads, exploring the foundational principles that underpin this essential engineering task.
Methods for Calculating Static Loads
Calculating static loads is a critical aspect of structural engineering, ensuring that buildings, bridges, and other structures can withstand the forces acting upon them without failure. There are several methods employed to determine these loads, each tailored to specific types of structures and loading conditions. One fundamental approach is the **Dead Load Calculation**, which involves summing the weights of all permanent components of the structure, including the building materials, finishes, and any fixed equipment. This method requires meticulous attention to detail, as it involves calculating the volume and density of various materials to derive their total weight. Another essential method is the **Live Load Calculation**, which accounts for temporary loads such as people, furniture, and movable equipment. These loads are typically specified by building codes and can vary significantly depending on the intended use of the space. For instance, a residential area would have a lower live load compared to a commercial or industrial setting. **Snow Load Calculations** are also crucial in regions where snowfall is significant, as the weight of accumulated snow can exert substantial pressure on roofs and other horizontal surfaces. In addition to these basic calculations, more complex structures often require **Wind Load Analysis** and **Seismic Load Analysis**. Wind loads are determined using wind speed data and the shape and orientation of the structure, while seismic loads are calculated based on the seismic activity of the region and the dynamic response of the structure to ground motion. These analyses often involve sophisticated computational models and simulations to accurately predict how the structure will behave under various loading scenarios. For more specialized structures like bridges, **Traffic Load Calculations** are necessary to account for the dynamic effects of moving vehicles. This involves considering factors such as vehicle weight distribution, speed, and frequency of passage. Similarly, in marine engineering, **Hydrostatic Load Calculations** are used to determine the pressure exerted by water on submerged or partially submerged structures like dams or offshore platforms. Advanced computational techniques such as **Finite Element Analysis (FEA)** have become increasingly popular for calculating static loads in complex geometries and materials. FEA allows engineers to model the structure in detail, apply various loads, and analyze the resulting stresses and deformations. This method provides a high degree of accuracy and is particularly useful for optimizing structural design and material usage. In summary, calculating static loads involves a combination of basic material weight calculations, adherence to building codes for live loads, and sophisticated analyses for wind, seismic, traffic, and hydrostatic loads. By employing these methods accurately and comprehensively, engineers can ensure that structures are designed to safely support all anticipated loads, thereby preventing failures and ensuring public safety. The integration of advanced computational tools further enhances the precision and reliability of these calculations, making modern structural engineering both robust and efficient.
Tools and Software Used in Analysis
When calculating and analyzing static loads, engineers and analysts rely on a variety of sophisticated tools and software to ensure accuracy and efficiency. At the forefront of these tools are finite element analysis (FEA) software packages such as ANSYS, ABAQUS, and NASTRAN. These programs allow users to model complex structures and simulate the effects of static loads, providing detailed insights into stress distributions, deformations, and potential failure points. Another crucial tool is computational fluid dynamics (CFD) software like OpenFOAM or Fluent, which is particularly useful for analyzing fluid-structure interactions that can influence static load calculations. In addition to these simulation tools, computer-aided design (CAD) software such as SolidWorks, Autodesk Inventor, and CATIA play a pivotal role in creating precise geometric models of structures. These models serve as the foundation for subsequent analyses, ensuring that the simulations accurately reflect real-world conditions. For data analysis and visualization, tools like MATLAB, Python libraries such as NumPy and Pandas, and specialized software like Excel or Tableau are indispensable. They enable users to process large datasets efficiently, perform statistical analyses, and present findings in a clear and compelling manner. Furthermore, specialized structural analysis software like ETABS for building structures or STAAD.Pro for general structural analysis offer tailored solutions that streamline the process of calculating static loads. These programs often include pre-built libraries of standard structural elements and automated calculation routines that save time while maintaining high levels of accuracy. Moreover, cloud-based platforms such as Onshape or Fusion 360 have revolutionized collaborative work by allowing multiple stakeholders to access and contribute to designs in real-time. This collaborative environment enhances the speed and quality of analysis by facilitating immediate feedback and iteration. In recent years, machine learning algorithms integrated into analytical software have begun to play a significant role in predicting structural behavior under static loads. Tools like TensorFlow or PyTorch can be used to develop predictive models that learn from historical data sets, thereby improving the accuracy of future analyses. Lastly, documentation and reporting tools such as LaTeX or Microsoft Word are essential for compiling comprehensive reports that detail the methodology, results, and conclusions drawn from static load analyses. These reports are critical for communicating findings to clients, peers, or regulatory bodies. In summary, the arsenal of tools and software available for analyzing static loads is diverse and powerful. By leveraging these technologies in conjunction with one another—combining simulation capabilities with data analysis tools and collaborative platforms—engineers can ensure that their calculations are both precise and reliable. This integrated approach not only enhances the accuracy of static load analyses but also accelerates the overall design process.
Common Challenges and Considerations
When calculating and analyzing static loads, several common challenges and considerations must be taken into account to ensure accurate and reliable results. One of the primary challenges is the complexity of structural systems, where multiple components interact in ways that can be difficult to model precisely. For instance, in buildings, the distribution of weight from floors to beams, columns, and foundations involves intricate load paths that require meticulous analysis to avoid underestimation or overestimation of stresses. Another significant consideration is the variability in material properties; different materials have unique strengths, densities, and elastic moduli that must be accurately accounted for to prevent miscalculations. Additionally, environmental factors such as temperature changes and humidity can affect material properties over time, necessitating dynamic rather than static assumptions in some cases. Geometric imperfections and construction tolerances also pose significant challenges. Even slight deviations from ideal shapes or dimensions can lead to unexpected stress concentrations or load redistributions. Furthermore, the presence of joints, connections, and other discontinuities introduces additional complexity due to potential slippage or deformation at these points. The choice of analytical method itself is another critical consideration; finite element analysis (FEA) might be necessary for complex geometries but may require substantial computational resources and expertise. Moreover, regulatory compliance is a crucial aspect; engineers must adhere to local building codes and standards which often specify minimum load requirements and safety factors. These standards can vary significantly between regions, adding an extra layer of complexity when working on international projects. Finally, the importance of validation through experimental testing cannot be overstated; theoretical calculations should ideally be verified against real-world data to ensure that assumptions made during analysis are valid. In summary, calculating and analyzing static loads involves navigating a myriad of challenges related to structural complexity, material variability, environmental influences, geometric imperfections, analytical methods, regulatory compliance, and validation. Addressing these considerations meticulously is essential for ensuring the safety and integrity of structures under various loading conditions. By understanding these challenges and taking them into account during the design process, engineers can produce more accurate analyses that translate into safer and more durable structures.
Applications and Real-World Examples of Static Loads
Static loads play a crucial role in various engineering disciplines and everyday life, influencing the design, safety, and functionality of structures and machines. This article delves into the applications and real-world examples of static loads, highlighting their significance across different fields. We will explore how structural engineers utilize static load analysis to ensure the stability and integrity of buildings, bridges, and other infrastructure. Additionally, we will examine the industrial and mechanical engineering uses of static loads, where they are essential for the design and operation of machinery and manufacturing processes. Furthermore, we will illustrate everyday examples of static load in action, demonstrating how these principles are applied in common scenarios such as furniture design and vehicle safety. By understanding these diverse applications, readers can appreciate the critical importance of static loads in maintaining safety, efficiency, and performance. Let us begin by examining the structural engineering applications of static loads, where precise calculations are paramount to preventing catastrophic failures and ensuring public safety.
Structural Engineering Applications
Structural engineering plays a pivotal role in ensuring the safety, durability, and functionality of various structures by meticulously managing static loads. Static loads, which are constant forces applied to a structure over time, are a critical consideration in the design and construction of buildings, bridges, dams, and other infrastructure. One of the most significant applications of structural engineering in handling static loads is in the design of high-rise buildings. Here, engineers must carefully calculate the weight of the building itself (dead load) along with any permanent fixtures and the anticipated weight of occupants and furniture (live load). This meticulous planning ensures that the foundation and structural elements can withstand these loads without compromising the integrity of the building. In bridge construction, structural engineers apply similar principles to account for static loads such as the weight of the bridge's materials and the expected traffic load. Advanced computational models and simulations are often used to predict how different types of static loads will affect the bridge's structure over its lifespan. For instance, in suspension bridges like the Golden Gate Bridge, engineers must balance the dead load of the bridge's components with the live load from vehicles and pedestrians while also considering environmental factors like wind resistance. Dams are another critical example where structural engineering's application in managing static loads is paramount. The primary static load on a dam is the hydrostatic pressure exerted by the water it holds back. Engineers use sophisticated analysis techniques to ensure that the dam's structure can resist this pressure without failing. Additionally, they consider other static loads such as the weight of the dam itself and any sediment or debris that may accumulate. In civil infrastructure projects like tunnels and underground structures, managing static loads is equally crucial. Here, engineers must account for the weight of overlying soil and rock (overburden) as well as any internal loads from construction materials. Advanced geotechnical engineering techniques are employed to stabilize these structures against collapse or deformation under these constant forces. Moreover, in industrial settings such as power plants and chemical processing facilities, structural engineers design structures to handle heavy machinery and equipment that impose significant static loads. These designs often involve complex calculations to ensure that foundations and support structures can bear these weights safely without risking structural failure. The application of structural engineering principles in managing static loads extends beyond traditional infrastructure projects. For example, in aerospace engineering, the design of aircraft and spacecraft involves careful consideration of static loads during launch and operation phases. Similarly, in offshore engineering, platforms and rigs must be designed to withstand the static loads imposed by their own weight as well as environmental forces like waves and currents. In conclusion, the role of structural engineering in managing static loads is indispensable across a wide range of applications. By leveraging advanced computational tools, rigorous testing protocols, and deep understanding of material properties and environmental conditions, structural engineers ensure that structures can safely support their intended loads over their entire lifespan. This meticulous attention to detail not only enhances safety but also optimizes resource usage and extends the service life of critical infrastructure.
Industrial and Mechanical Engineering Uses
Industrial and mechanical engineering play pivotal roles in the design, development, and implementation of systems that withstand static loads, ensuring the safety, efficiency, and reliability of various structures and machines. In industrial settings, static loads are a critical consideration for engineers designing buildings, bridges, and other infrastructure. For instance, the structural integrity of a skyscraper depends on its ability to support the weight of its own materials (dead load) as well as the weight of occupants and furniture (live load). Mechanical engineers must meticulously calculate these loads to ensure that the building's foundation, beams, and columns can bear the stress without compromising safety. In mechanical engineering, static loads are equally important in the design of machinery and equipment. For example, in the automotive industry, engineers must consider the static loads on vehicle components such as axles and suspension systems to ensure they can handle the weight of the vehicle and its payload. Similarly, in aerospace engineering, the static loads on aircraft components like wings and landing gear are crucial for maintaining structural integrity during takeoff, landing, and flight. Real-world examples abound in manufacturing facilities where machinery like cranes and hoists are designed to handle heavy static loads. These machines must be engineered to lift and hold significant weights without failing, which requires precise calculations of stress and strain. Additionally, in renewable energy sectors such as wind power, mechanical engineers design wind turbines that must withstand static loads from their own weight as well as dynamic loads from wind forces. The application of static load principles extends to consumer products as well. For instance, furniture manufacturers must ensure that their products can support the intended weight without collapsing or deforming excessively. This involves rigorous testing under static load conditions to validate design assumptions. Moreover, advancements in materials science have allowed engineers to develop new materials with improved strength-to-weight ratios, enabling more efficient designs that can handle higher static loads while minimizing material usage. This is particularly evident in aerospace and automotive industries where lightweight yet strong materials like carbon fiber are increasingly used. In summary, understanding and managing static loads are fundamental aspects of industrial and mechanical engineering. By accurately calculating and designing for these loads, engineers can create safe, efficient, and reliable systems that perform optimally under various conditions. This expertise is crucial across a wide range of applications, from large-scale infrastructure projects to everyday consumer products, highlighting the indispensable role of industrial and mechanical engineering in modern society.
Everyday Examples of Static Load in Action
Static loads are ubiquitous in our daily lives, influencing the design and functionality of various structures and objects we interact with. One of the most common examples is the weight-bearing capacity of buildings. When architects design a building, they must consider the static load imposed by the weight of the structure itself, including walls, floors, and roofs, as well as the weight of occupants and furniture. This ensures that the foundation and support beams can withstand these constant forces without collapsing or deforming excessively. Similarly, bridges are engineered to handle static loads from their own weight and the anticipated weight of vehicles that will cross them, ensuring they remain stable and secure over time. In residential settings, furniture like beds and chairs are designed to support specific static loads based on average human weight. For instance, a bed frame is constructed to bear the combined weight of its occupants without sagging or breaking. Even household items such as shelves and cabinets are built with static loads in mind; they are designed to hold a certain amount of weight from books, dishes, or other stored items without collapsing. Public infrastructure also relies heavily on understanding static loads. For example, roads are built to withstand the static load of parked vehicles and the cumulative weight of traffic over time. This involves selecting materials and designing roadbeds that can distribute these loads evenly to prevent cracking and deterioration. In urban planning, sidewalks and pedestrian paths must be able to support the static load of foot traffic without showing signs of wear prematurely. In industrial settings, machinery and equipment are often subjected to static loads during operation. For example, a crane lifting heavy loads must be designed to handle both the dynamic forces during lifting and the static forces when the load is stationary. Similarly, storage racks in warehouses are engineered to bear the static load of stacked goods without risking collapse or structural failure. Even in consumer products, static loads play a crucial role. For instance, luggage racks on airplanes are designed to support the combined weight of multiple suitcases without compromising safety or structural integrity. In sports equipment, items like gymnastics bars and climbing walls must be able to withstand the static load of athletes' weights during use. Understanding and managing static loads is essential for ensuring safety, durability, and efficiency across various sectors. By accurately calculating and accommodating these loads, engineers can create structures and products that are reliable, long-lasting, and capable of performing their intended functions without failure. This meticulous attention to detail in design helps prevent accidents, reduces maintenance costs, and enhances overall user experience. In essence, recognizing the impact of static loads in everyday examples underscores their critical role in modern engineering and design practices.