What Is Thermal Equilibrium
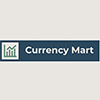
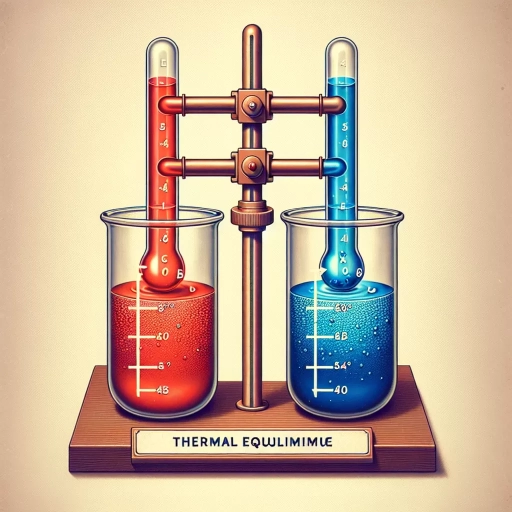
Thermal equilibrium is a fundamental concept in physics that describes the state where the temperature is uniform throughout a system or between systems in contact. This state is crucial for understanding various natural and engineered processes, from the simplest everyday phenomena to complex industrial applications. In this article, we will delve into the intricacies of thermal equilibrium, starting with a comprehensive explanation of what it entails and how it is achieved. We will explore the key concepts related to thermal equilibrium, including the principles of heat transfer, the zeroth law of thermodynamics, and the role of temperature in defining equilibrium. Additionally, we will examine the practical applications and examples of thermal equilibrium, highlighting its significance in fields such as engineering, chemistry, and environmental science. By understanding these aspects, readers will gain a deeper appreciation for the importance of thermal equilibrium in both theoretical and practical contexts. Let us begin by **Understanding Thermal Equilibrium**.
Understanding Thermal Equilibrium
Understanding thermal equilibrium is a fundamental concept in physics that underpins various natural and technological processes. This article delves into the intricacies of thermal equilibrium, providing a comprehensive overview that is both informative and engaging. We begin by defining thermal equilibrium and exploring its basic principles, which form the cornerstone of our understanding. This foundational knowledge is crucial for grasping how systems interact and stabilize at a uniform temperature. Next, we examine the conditions necessary for achieving thermal equilibrium, highlighting the critical factors that must be met for this state to occur. Finally, we discuss the importance of thermal equilibrium in various fields, from engineering and chemistry to biology and environmental science, illustrating its practical applications and significance. By understanding these aspects, readers will gain a deeper appreciation for the role thermal equilibrium plays in maintaining balance and stability across diverse systems. Let us start by defining thermal equilibrium and its basic principles, which will serve as the foundation for our exploration.
Definition and Basic Principles
**Definition and Basic Principles** Thermal equilibrium is a fundamental concept in thermodynamics, describing the state where the temperature is uniform throughout a system or between systems in contact. This equilibrium is achieved when there is no net heat transfer between the systems, indicating that the temperature has stabilized and there are no longer any thermal gradients. The definition hinges on the principle that heat transfer occurs from regions of higher temperature to those of lower temperature until thermal balance is reached. At its core, thermal equilibrium is governed by the zeroth law of thermodynamics. This law states that if two systems are each in thermal equilibrium with a third system, then they are also in thermal equilibrium with each other. This allows for the definition of a temperature scale, such as the Celsius or Kelvin scale, which quantifies the thermal state of a system. When two systems are brought into contact, heat flows from the hotter system to the cooler one until their temperatures equalize, marking the attainment of thermal equilibrium. The basic principles underlying thermal equilibrium include the conservation of energy and the concept of entropy. According to the first law of thermodynamics, energy cannot be created or destroyed, only converted from one form to another. In the context of thermal equilibrium, this means that any heat energy transferred from one system to another results in an equal and opposite change in their internal energies. Entropy, a measure of disorder or randomness, also plays a crucial role. As systems approach thermal equilibrium, their total entropy tends to maximize, reflecting the natural tendency towards disorder and uniformity. Understanding these principles is essential for predicting and analyzing various physical phenomena. For instance, in engineering applications, maintaining thermal equilibrium is critical for ensuring the optimal performance and longevity of machinery. In biological systems, thermal equilibrium helps regulate body temperature, which is vital for metabolic processes and overall health. Furthermore, in environmental science, studying thermal equilibrium aids in understanding climate dynamics and the Earth's energy balance. In practical terms, achieving thermal equilibrium can be observed in everyday life. For example, when a hot cup of coffee is left on a table, it eventually cools down to room temperature due to heat transfer with its surroundings. Similarly, when two objects at different temperatures are placed in contact, they will eventually reach a common temperature as heat flows from the hotter object to the cooler one. In summary, thermal equilibrium is a state characterized by uniform temperature and no net heat transfer. It is founded on the zeroth law of thermodynamics and governed by principles such as energy conservation and entropy maximization. Understanding these concepts is pivotal for various scientific and engineering applications, as well as for appreciating natural phenomena that occur around us. By recognizing how thermal equilibrium operates, we can better comprehend and predict the behavior of systems in diverse contexts.
Conditions for Achieving Thermal Equilibrium
Achieving thermal equilibrium is a fundamental concept in thermodynamics, and it involves specific conditions that must be met for a system to reach this state. Thermal equilibrium occurs when the temperature is uniform throughout a system or between systems in contact with each other. The primary condition for achieving thermal equilibrium is that there must be no net heat transfer between the system and its surroundings or among different parts of the system. This means that the system has reached a stable temperature, where the rate of heat flow into the system equals the rate of heat flow out of the system. Another crucial condition is that the system must be isolated from external influences that could cause temperature changes. This includes ensuring that there are no external heat sources or sinks and that the system is well-insulated to prevent any significant heat exchange with the environment. Additionally, all parts of the system must be in contact with each other, either directly or indirectly, to allow for the free exchange of heat energy until a uniform temperature is achieved. The process of reaching thermal equilibrium can be influenced by several factors, including the specific heat capacity of the materials involved, the mass of the components, and the initial temperature differences. For instance, materials with higher specific heat capacities require more energy to change their temperature by a given amount, which can slow down the process of achieving equilibrium. Similarly, larger masses take longer to reach equilibrium due to their greater thermal inertia. In practical terms, achieving thermal equilibrium is often facilitated by using materials with high thermal conductivity, such as metals, which allow for efficient heat transfer. Stirring or mixing can also accelerate the process by ensuring uniform contact and minimizing temperature gradients within the system. Understanding these conditions is essential in various fields such as engineering, chemistry, and physics, where precise control over temperature is critical for accurate measurements and reliable operations. Moreover, the concept of thermal equilibrium extends beyond simple systems to complex scenarios involving multiple phases and components. For example, in a mixture of liquids and gases, each phase must reach thermal equilibrium with the others for the entire system to be considered in thermal equilibrium. This requires careful consideration of phase transitions and the thermodynamic properties of each component. In summary, achieving thermal equilibrium involves ensuring no net heat transfer, isolating the system from external influences, allowing free exchange of heat among all parts of the system, and considering material properties and system configurations. By understanding these conditions, one can effectively manage and predict the behavior of systems in various scientific and engineering contexts. This foundational knowledge is pivotal for advancing our understanding of thermodynamic principles and their applications in real-world scenarios.
Importance in Various Fields
Thermal equilibrium, a fundamental concept in thermodynamics, holds significant importance across various fields due to its role in understanding and predicting the behavior of systems under different thermal conditions. In **engineering**, thermal equilibrium is crucial for designing efficient heat transfer systems, such as refrigeration units, air conditioning systems, and power plants. Engineers rely on the principles of thermal equilibrium to ensure that these systems operate at optimal temperatures, thereby maximizing their performance and minimizing energy consumption. For instance, in the automotive industry, understanding thermal equilibrium helps in the design of cooling systems for engines, which is essential for maintaining engine efficiency and longevity. In **medicine**, thermal equilibrium plays a vital role in medical imaging techniques like MRI (Magnetic Resonance Imaging) and PET (Positron Emission Tomography). These imaging technologies require precise control over temperature to ensure accurate imaging and diagnosis. Additionally, thermal equilibrium is important in the development of medical devices such as thermometers and temperature sensors used in patient monitoring. In **environmental science**, understanding thermal equilibrium helps scientists study climate dynamics and predict weather patterns. The Earth's atmosphere is in a state of thermal equilibrium with the sun and space, and disruptions to this balance can lead to climate change. By analyzing these equilibria, researchers can better understand global warming trends and develop strategies to mitigate its effects. In **chemistry**, thermal equilibrium is essential for understanding chemical reactions and their kinetics. The concept of equilibrium constant (Kc or Kp) is directly related to thermal equilibrium, as it describes the ratio of reactants to products at a given temperature. This knowledge is critical for optimizing reaction conditions in industrial processes, such as the Haber-Bosch process for ammonia production. In **physics**, thermal equilibrium underpins many theoretical models, including the Boltzmann distribution, which describes the statistical distribution of particles in a system at thermal equilibrium. This distribution is fundamental to understanding phenomena such as Brownian motion and the behavior of gases. In **biological systems**, maintaining thermal equilibrium is vital for life processes. Organisms have intricate mechanisms to regulate their internal temperature despite external changes, ensuring that biochemical reactions occur within an optimal range. For example, humans have a complex thermoregulatory system involving sweat glands, blood circulation adjustments, and metabolic rate changes to maintain a stable body temperature. Lastly, in **space exploration**, understanding thermal equilibrium is crucial for designing spacecraft and habitats that can withstand extreme temperature variations in space environments. Spacecraft must be able to maintain stable internal temperatures despite external conditions ranging from the freezing cold of space to the intense heat generated during re-entry into Earth's atmosphere. In summary, the concept of thermal equilibrium is not just a theoretical construct but has far-reaching practical implications across diverse fields. Its importance lies in its ability to explain and predict how systems behave under different thermal conditions, thereby enabling advancements in technology, medicine, environmental science, chemistry, physics, biology, and space exploration. Understanding thermal equilibrium is essential for optimizing performance, ensuring safety, and advancing our knowledge of the world around us.
Key Concepts Related to Thermal Equilibrium
Understanding thermal equilibrium is crucial for grasping the fundamental principles of thermodynamics. This concept is intricately linked with several key ideas that collectively provide a comprehensive view of how systems interact and stabilize. First, **Temperature and Heat Transfer** play a pivotal role in achieving thermal equilibrium. Temperature, a measure of the average kinetic energy of particles, dictates the direction of heat flow, while heat transfer mechanisms such as conduction, convection, and radiation facilitate the exchange of energy between systems until they reach a uniform temperature. Second, **Thermodynamic Systems and Processes** are essential for analyzing how energy transformations occur within and between systems. These processes, whether isothermal, adiabatic, or isobaric, influence the path to equilibrium. Lastly, **Equilibrium States and Stability** ensure that once a system reaches thermal equilibrium, it remains stable unless external conditions change. This stability is a result of the balance between internal energy and the environment. By delving into these interconnected concepts, we can gain a deeper understanding of how thermal equilibrium is achieved and maintained. Let's begin by exploring the critical role of **Temperature and Heat Transfer** in this process.
Temperature and Heat Transfer
Temperature and heat transfer are fundamental concepts that underpin the understanding of thermal equilibrium. Temperature, a measure of the average kinetic energy of particles in a substance, is crucial because it indicates the thermal state of a system. When two systems are in contact, heat transfer occurs until they reach the same temperature, a state known as thermal equilibrium. This process is governed by the laws of thermodynamics, particularly the zeroth law, which states that if two systems are each in thermal equilibrium with a third system, then they are also in thermal equilibrium with each other. Heat transfer itself can occur through three primary mechanisms: conduction, convection, and radiation. Conduction involves direct contact between particles and is most efficient in solids where atoms are closely packed. Convection relies on the movement of fluids to transfer heat, often enhanced by natural or forced circulation. Radiation, on the other hand, involves the transfer of energy through electromagnetic waves and can occur across a vacuum. Understanding these mechanisms is essential for predicting how systems will reach thermal equilibrium. In practical terms, heat transfer plays a critical role in various engineering and scientific applications. For instance, in electronics cooling, efficient heat transfer is necessary to prevent overheating and ensure the longevity of components. Similarly, in climate science, understanding global heat transfer patterns helps predict weather and climate phenomena. The concept of thermal equilibrium also has biological implications; for example, the human body maintains a stable internal temperature despite external changes through complex heat transfer processes. The study of temperature and heat transfer also delves into the properties of materials and their thermal conductivities. Materials with high thermal conductivity, such as metals, facilitate rapid heat transfer, while insulators like foam or fiberglass hinder it. This knowledge is pivotal in designing thermal insulation for buildings or developing advanced materials for aerospace applications. Furthermore, the principles of temperature and heat transfer are integral to understanding phase changes and the behavior of substances at different temperatures. For example, when a substance reaches its boiling point, it undergoes a phase transition from liquid to gas, absorbing latent heat without changing temperature until the transition is complete. This phenomenon is crucial in processes like refrigeration and steam power generation. In conclusion, temperature and heat transfer are key components of thermal equilibrium. By grasping these concepts—how temperature measures thermal energy, how heat is transferred through conduction, convection, and radiation, and how these processes affect various systems—we can better understand and predict the behavior of systems as they approach thermal equilibrium. This foundational knowledge has far-reaching implications across multiple disciplines, from engineering and climate science to biology and materials science, highlighting the importance of these principles in both theoretical and practical contexts.
Thermodynamic Systems and Processes
Thermodynamic systems and processes are fundamental concepts in understanding thermal equilibrium. A thermodynamic system is a region of space where changes due to energy transfer or work are studied. These systems can be classified into three main types: isolated, closed, and open. An isolated system does not exchange matter or energy with its surroundings, a closed system exchanges energy but not matter, and an open system exchanges both energy and matter. The behavior of these systems is governed by the laws of thermodynamics, particularly the zeroth law, which defines thermal equilibrium. In thermal equilibrium, the temperature is uniform throughout the system and its surroundings. This state is achieved when there are no net heat flows between the system and its environment. Thermodynamic processes describe how a system changes from one state to another. Key processes include isothermal (constant temperature), adiabatic (no heat transfer), isobaric (constant pressure), and isochoric (constant volume). Each process has specific implications for the system's energy and entropy changes. For instance, an isothermal expansion involves a system expanding while maintaining a constant temperature, often achieved by heat transfer to or from the surroundings. This process is crucial in understanding how gases behave in real-world applications, such as in engines and refrigeration systems. On the other hand, an adiabatic process occurs without any heat exchange, leading to changes in temperature and pressure as the system expands or contracts. The concept of entropy is also pivotal in thermodynamic processes. Entropy measures the disorder or randomness of a system. According to the second law of thermodynamics, entropy tends to increase over time in an isolated system, reflecting the direction of spontaneous processes. In thermal equilibrium, entropy is maximized, indicating that the system has reached its most stable state. Understanding these thermodynamic systems and processes is essential for various technological and scientific applications. For example, in power generation, thermodynamic cycles like the Carnot cycle and Rankine cycle are used to convert thermal energy into mechanical work efficiently. Similarly, in refrigeration and air conditioning, thermodynamic principles guide the design of systems that maintain desired temperatures by transferring heat from one location to another. In summary, thermodynamic systems and processes provide a comprehensive framework for analyzing and predicting the behavior of energy and matter under different conditions. By grasping these concepts, scientists and engineers can design more efficient and sustainable technologies that rely on the principles of thermal equilibrium. This understanding is crucial for advancing fields such as energy production, storage, and utilization, as well as environmental science and engineering.
Equilibrium States and Stability
In the context of thermal equilibrium, understanding equilibrium states and stability is crucial for grasping the fundamental principles that govern the behavior of physical systems. An equilibrium state is a condition where the macroscopic properties of a system remain constant over time, indicating that the system has reached a balance with its surroundings. This balance can be achieved in various forms, such as mechanical, thermal, or chemical equilibrium. For instance, in thermal equilibrium, the temperature throughout the system and its surroundings is uniform, meaning there is no net heat transfer between them. Stability in an equilibrium state refers to the system's ability to return to its original state after being subjected to a small perturbation or disturbance. A stable equilibrium is one where the system tends to return to its original state after any minor disruption. Conversely, an unstable equilibrium occurs when even a slight disturbance causes the system to move away from its original state. For example, consider a ball placed at the top of a hill; this represents an unstable equilibrium because any slight push will cause the ball to roll down the hill. On the other hand, a ball at the bottom of a valley represents a stable equilibrium since it will return to this position if slightly displaced. The concept of stability is closely related to potential energy and the shape of the potential energy landscape. In stable equilibria, the potential energy is at a minimum, while in unstable equilibria, it is at a maximum. This can be visualized using the analogy of hills and valleys: stable equilibria are like valleys where potential energy is low, and unstable equilibria are like hilltops where potential energy is high. In thermal systems, stability can be influenced by factors such as temperature gradients and heat transfer mechanisms. For instance, in a thermally isolated system reaching thermal equilibrium, internal temperature differences are eliminated through heat transfer until all parts of the system have the same temperature. However, if external conditions change (e.g., if one part of the system is suddenly heated), the stability of this equilibrium can be disrupted until a new equilibrium state is reached. Understanding these concepts is essential for predicting and controlling the behavior of various physical and engineering systems. In engineering applications, maintaining stable thermal equilibria is critical for ensuring efficient operation and longevity of equipment. For example, in electronic devices, maintaining stable temperatures helps prevent overheating and ensures reliable performance. Moreover, the study of equilibrium states and stability extends beyond simple mechanical or thermal systems to complex biological and chemical systems. In biological contexts, homeostasis—the ability of living organisms to maintain internal stability despite changes in external conditions—relied heavily on principles of equilibrium and stability. Similarly, in chemical reactions, understanding the stability of reactants and products helps predict reaction outcomes and optimize reaction conditions. In conclusion, equilibrium states and stability form the backbone of understanding how physical systems behave under different conditions. By recognizing these principles, scientists and engineers can design more efficient systems, predict system behavior under various perturbations, and ensure optimal performance across a wide range of applications. This foundational knowledge is pivotal for advancing our understanding of thermal equilibrium and its broader implications in science and technology.
Applications and Examples of Thermal Equilibrium
Thermal equilibrium, a fundamental concept in thermodynamics, is the state where the temperature is uniform throughout a system or between systems in contact. This principle has far-reaching implications across various domains, making it a cornerstone of both theoretical and practical applications. In industrial and engineering contexts, thermal equilibrium plays a crucial role in the design and operation of machinery, ensuring optimal performance and efficiency. For instance, heat exchangers rely on achieving thermal equilibrium to transfer heat effectively between different fluids. In biological and environmental contexts, thermal equilibrium is essential for understanding the balance of ecosystems and the physiological processes of living organisms. For example, the Earth's climate system is in a delicate state of thermal equilibrium, influenced by factors such as solar radiation and greenhouse gases. This balance is vital for maintaining life-supporting conditions on our planet. Everyday life is also replete with examples of thermal equilibrium. From the heating and cooling systems in our homes to the thermoses that keep our beverages at a consistent temperature, these technologies all depend on the principles of thermal equilibrium to function effectively. Understanding these applications not only enhances our appreciation for the science behind everyday conveniences but also underscores the importance of thermal equilibrium in maintaining our quality of life. Transitioning to industrial and engineering applications, we delve into how this concept is harnessed to drive innovation and efficiency in various sectors.
Industrial and Engineering Applications
In the realm of industrial and engineering applications, the concept of thermal equilibrium plays a pivotal role in ensuring the efficiency, safety, and reliability of various systems. Thermal equilibrium, where the temperature is uniform throughout a system or between systems in contact, is crucial for maintaining optimal operating conditions. For instance, in power plants, thermal equilibrium is essential for the efficient operation of heat exchangers and boilers. These components must operate at specific temperatures to maximize energy conversion and minimize wear and tear. Any deviation from thermal equilibrium can lead to reduced efficiency, increased maintenance costs, and potential safety hazards. In the automotive industry, thermal equilibrium is vital for engine performance and longevity. Modern engines are designed to operate within a narrow temperature range to achieve optimal fuel efficiency and minimize emissions. Cooling systems, including radiators and heat exchangers, are engineered to maintain thermal equilibrium between the engine and the environment, thereby preventing overheating which could cause engine damage. Aerospace engineering also heavily relies on thermal equilibrium. Spacecraft and satellites must be designed to maintain stable temperatures despite extreme environmental conditions. This involves using advanced materials and insulation techniques to ensure that electronic components operate within their specified temperature ranges, thereby ensuring reliable performance in space missions. In chemical processing plants, thermal equilibrium is critical for controlling reaction rates and product quality. Chemical reactions often require precise temperature control to achieve desired outcomes, and deviations from thermal equilibrium can result in suboptimal yields or even dangerous conditions such as explosions. Furthermore, in HVAC (Heating, Ventilation, and Air Conditioning) systems, maintaining thermal equilibrium is essential for providing comfortable indoor environments while optimizing energy consumption. These systems are designed to balance heat transfer between different parts of a building to maintain a consistent temperature, thereby enhancing occupant comfort and reducing energy costs. Additionally, in medical devices such as MRI machines and laser equipment, precise temperature control is necessary to ensure accurate diagnostics and treatments. These devices often require sophisticated cooling systems to maintain thermal equilibrium during operation, which is critical for their functionality and patient safety. In summary, industrial and engineering applications underscore the importance of achieving and maintaining thermal equilibrium across diverse fields. By ensuring uniform temperatures within systems or between interacting components, engineers can enhance performance, safety, efficiency, and reliability—ultimately driving innovation and progress in various sectors.
Biological and Environmental Contexts
In the realm of biological and environmental contexts, thermal equilibrium plays a pivotal role in maintaining the delicate balance of ecosystems and the health of living organisms. Thermal equilibrium, where the temperature of a system remains constant over time due to balanced heat exchange with its surroundings, is crucial for various biological processes. For instance, in humans, the body's ability to maintain a stable internal temperature around 37°C (98.6°F) is essential for metabolic functions, enzyme activity, and overall physiological well-being. This homeostatic regulation involves intricate mechanisms such as sweating, shivering, and vasodilation or vasoconstriction to ensure that the body's temperature remains within a narrow range despite external environmental changes. In ecosystems, thermal equilibrium influences the distribution and behavior of species. For example, many aquatic organisms have optimal temperature ranges for survival and reproduction. Fish like salmon migrate to cooler waters during spawning to ensure the survival of their offspring, while coral reefs thrive in warmer tropical waters but are vulnerable to bleaching when temperatures exceed their tolerance limits. Similarly, terrestrial ecosystems such as forests and grasslands have species that are adapted to specific temperature regimes; changes in these regimes due to climate change can disrupt these ecosystems by altering the distribution of plants and animals. Thermal equilibrium also impacts agricultural productivity. Crops have optimal temperature ranges for growth and yield. For instance, maize (corn) grows best in temperatures between 20°C and 30°C (68°F to 86°F), while wheat thrives in cooler temperatures. Understanding and managing thermal equilibrium in agricultural settings through practices like irrigation and mulching can significantly enhance crop yields and resilience to climate variability. Furthermore, environmental phenomena such as global climate patterns are influenced by thermal equilibrium. The Earth's climate system is in a state of dynamic thermal equilibrium with the Sun and space. The balance between incoming solar radiation and outgoing infrared radiation determines the Earth's average temperature. Human activities that alter this balance, such as the emission of greenhouse gases, can disrupt this equilibrium, leading to global warming and associated climate change impacts. In addition to these biological and ecological contexts, thermal equilibrium has practical applications in environmental monitoring and conservation. For example, thermometers and other temperature-sensing devices are used to monitor water quality in rivers and lakes, helping to detect changes that could indicate pollution or other environmental stressors. In conservation efforts, understanding thermal equilibrium helps in designing habitats for endangered species; for instance, creating artificial nests with optimal thermal conditions can enhance the survival rates of species like sea turtles. In conclusion, thermal equilibrium is not just a physical concept but has profound implications for biological systems and environmental health. Its role in maintaining homeostasis in organisms, influencing species distribution in ecosystems, impacting agricultural productivity, shaping global climate patterns, and aiding in environmental monitoring underscores its importance across diverse contexts. As we continue to navigate the complexities of climate change and environmental sustainability, understanding and managing thermal equilibrium will remain a critical aspect of preserving life on Earth.
Everyday Life Examples
Thermal equilibrium is a fundamental concept in physics that manifests in various aspects of everyday life, making it both relevant and relatable. One of the most common examples is the regulation of body temperature in humans. The human body maintains a constant internal temperature of approximately 37°C (98.6°F) through a delicate balance of heat production and dissipation, ensuring that it remains in thermal equilibrium with its surroundings. This balance is crucial for the proper functioning of metabolic processes and overall health. In cooking, thermal equilibrium plays a key role. When you boil water, it eventually reaches a steady state where the rate of heat input from the stove equals the rate of heat loss to the surroundings, achieving thermal equilibrium at 100°C (212°F) at sea level. Similarly, when baking a cake, the oven is set to a specific temperature to ensure that the cake cooks uniformly and reaches thermal equilibrium with the oven environment. Another everyday example is found in refrigeration systems. Your refrigerator operates by maintaining a lower temperature inside compared to the outside environment. This is achieved through continuous heat transfer from the interior to the exterior, ensuring that the contents inside remain at a stable, cooler temperature in thermal equilibrium with the refrigerated space. Thermal equilibrium is also evident in climate control systems in buildings. Air conditioning and heating systems work to maintain a consistent indoor temperature despite external weather conditions. By balancing heat gain and loss, these systems keep the indoor environment in thermal equilibrium, providing a comfortable living or working space. Furthermore, thermal equilibrium is crucial in automotive cooling systems. The radiator in a car engine helps to dissipate excess heat generated during combustion, ensuring that the engine operates within a safe temperature range. This process involves achieving thermal equilibrium between the engine coolant and the surrounding air, preventing overheating and potential damage to the engine. In addition, thermal equilibrium is essential for maintaining the quality of perishable goods. For instance, supermarkets use chilled display cases to keep dairy products and meats at a consistent refrigerated temperature. This ensures that these items remain in thermal equilibrium with their surroundings, extending their shelf life and preventing spoilage. Lastly, thermal equilibrium is observed in natural phenomena such as the Earth's climate system. The Earth's surface temperature is regulated by the balance between incoming solar radiation and outgoing infrared radiation, maintaining an average global temperature that supports life as we know it. This balance is a prime example of thermal equilibrium on a large scale. These examples illustrate how thermal equilibrium is not just a theoretical concept but an integral part of our daily lives, influencing everything from our health and comfort to the functioning of various technologies and natural systems. Understanding thermal equilibrium helps us appreciate the intricate balance that governs many aspects of our world.