What Is The Particle Model Of Matter
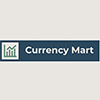
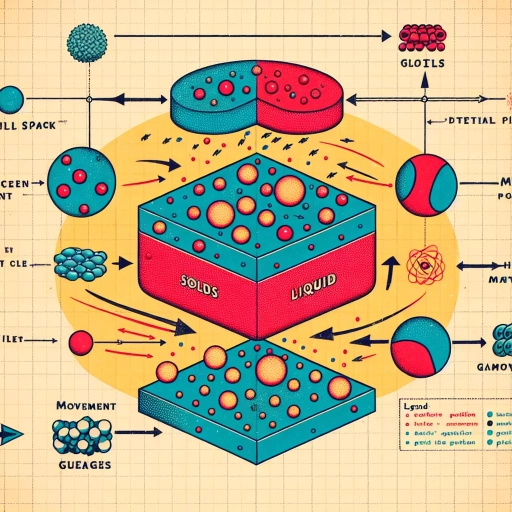
The particle model of matter is a fundamental concept in physics that explains the composition and behavior of substances at the atomic and subatomic level. This model posits that all matter is composed of tiny, indivisible particles called atoms, which in turn are made up of even smaller particles such as protons, neutrons, and electrons. Understanding the particle model is crucial for grasping various scientific principles and technological applications. In this article, we will delve into the core aspects of the particle model, starting with an **Introduction to the Particle Model of Matter**, where we will explore its historical development and basic principles. We will then examine the **Components and Structure of the Particle Model**, detailing the roles and interactions of atoms and their constituent particles. Finally, we will discuss the **Applications and Implications of the Particle Model**, highlighting how this concept influences fields such as chemistry, materials science, and engineering. By the end of this journey, readers will have a comprehensive understanding of how the particle model shapes our understanding of the world around us. Let us begin by diving into the **Introduction to the Particle Model of Matter**.
Introduction to the Particle Model of Matter
The Particle Model of Matter is a foundational concept in modern physics, offering a profound understanding of the structure and behavior of materials at the atomic and subatomic level. This model has evolved significantly over time, shaped by historical developments that have refined our understanding of matter. The article delves into the **Historical Development of the Model**, tracing the milestones from ancient Greek philosophers to modern-day scientists who have contributed to its formulation. It also explores the **Key Principles and Assumptions** that underpin the model, including the idea that matter is composed of discrete particles and the rules governing their interactions. Furthermore, it highlights the **Importance in Modern Science**, demonstrating how this model is crucial for advancements in fields such as chemistry, physics, and engineering. By understanding these aspects, readers can appreciate the comprehensive nature of the Particle Model and its indispensable role in scientific inquiry. Transitioning to the historical development, we will examine how this model has been shaped over centuries, laying the groundwork for its current form and significance.
Historical Development of the Model
The historical development of the particle model of matter is a rich and evolving narrative that spans thousands of years, reflecting humanity's relentless pursuit of understanding the fundamental nature of reality. This journey began with ancient philosophers such as Democritus, who around 450 BCE proposed the concept of atoms as indivisible, eternal particles that make up all matter. This early atomic theory, though rudimentary, laid the groundwork for later scientific inquiry. In the 17th and 18th centuries, scientists like Robert Boyle and Antoine Lavoisier contributed significantly to the field by establishing the laws of chemical reactions and identifying elements, which further solidified the idea that matter is composed of discrete particles. The 19th century saw a major leap forward with the work of John Dalton, who in 1803 formulated the modern atomic theory. Dalton's theory posited that elements are composed of small, indivisible particles called atoms, and that compounds are formed when atoms of different elements combine in whole number ratios. This theory was later supported by the kinetic theory of gases developed by Ludwig Boltzmann and others, which described gases as collections of tiny particles in constant motion. The early 20th century brought about a revolutionary shift with the discovery of subatomic particles. J.J. Thomson's discovery of the electron in 1897 revealed that atoms were not indivisible but rather composed of smaller particles. Ernest Rutherford's famous gold foil experiment in 1909 led to the development of the nuclear model of the atom, where protons reside in a central nucleus surrounded by electrons. Niels Bohr's subsequent work introduced energy levels and electron shells, providing a more detailed understanding of atomic structure. The discovery of the neutron by James Chadwick in 1932 completed the basic picture of the atom as we know it today: a nucleus containing protons and neutrons, surrounded by electrons. This foundational knowledge has been continually refined through advancements in quantum mechanics and particle physics. The development of the Standard Model in particle physics during the latter half of the 20th century further elucidated the nature of subatomic particles, including quarks and leptons, which are now recognized as the building blocks of protons and neutrons. Throughout this historical trajectory, each discovery has built upon previous findings, gradually unveiling a more precise and comprehensive understanding of matter at its most fundamental level. The particle model of matter, as it stands today, is a testament to human curiosity and scientific ingenuity, providing a robust framework for explaining a wide range of phenomena from chemical reactions to the structure of materials. This model continues to guide research in various fields, from chemistry and physics to materials science and engineering, underscoring its significance as a cornerstone of modern scientific understanding.
Key Principles and Assumptions
The particle model of matter, a foundational concept in physics and chemistry, is built upon several key principles and assumptions that underpin our understanding of the structure and behavior of matter. At its core, this model posits that all matter is composed of tiny, indivisible particles known as atoms or molecules. One of the primary assumptions is the **atomic hypothesis**, which states that atoms are the fundamental building blocks of matter and cannot be created or destroyed in chemical reactions. This idea, first proposed by John Dalton in the early 19th century, forms the basis for modern atomic theory. Another crucial principle is the **law of conservation of mass**, which asserts that the total mass of a closed system remains constant over time. This law supports the particle model by indicating that matter can change forms but not be created or destroyed. The **law of definite proportions** further reinforces this model by stating that a chemical compound always contains its component elements in fixed ratio by mass, regardless of its source or method of preparation. The particle model also relies on the concept of **discrete particles**, meaning that matter is not continuous but rather consists of distinct, countable units. This contrasts with the older continuous or fluid model of matter, where substances were thought to blend smoothly into one another. The discrete nature of particles is evident in phenomena such as Brownian motion, where the random movement of particles suspended in a fluid can be observed. Additionally, the **kinetic molecular theory** plays a significant role in the particle model. This theory describes the behavior of gases and assumes that gas molecules are in constant random motion, colliding with each other and the walls of their container. This theory explains properties such as pressure and temperature in terms of molecular interactions, further solidifying the particle model's validity. The **periodic table**, which organizes elements based on their atomic number and recurring chemical properties, is another cornerstone of the particle model. It illustrates how elements with similar properties recur at regular intervals when arranged by increasing atomic number, reinforcing the idea that atoms are the fundamental units of matter. In summary, the particle model of matter is grounded in several key principles and assumptions: the atomic hypothesis, laws of conservation and definite proportions, discrete particles, kinetic molecular theory, and the periodic table. These concepts collectively provide a robust framework for understanding how matter behaves at its most fundamental level, enabling scientists to predict and explain a wide range of physical and chemical phenomena. By grasping these principles, we gain insight into the intricate structure and dynamic interactions that govern our physical world.
Importance in Modern Science
In the realm of modern science, the particle model of matter stands as a cornerstone, underpinning our understanding of the physical world. This model, which posits that matter is composed of discrete particles such as atoms and molecules, is pivotal for several reasons. Firstly, it provides a fundamental framework for explaining various physical phenomena. By recognizing that matter is not continuous but rather composed of tiny, indivisible units, scientists can elucidate processes like chemical reactions, phase transitions, and the behavior of gases. For instance, the kinetic theory of gases, which is based on the particle model, allows us to understand how temperature and pressure affect gas behavior, a concept crucial in fields such as chemistry, physics, and engineering. Moreover, the particle model has been instrumental in advancing technological innovations. In materials science, understanding the atomic structure of materials has led to the development of new materials with tailored properties, such as nanomaterials and semiconductors. These advancements have revolutionized industries ranging from electronics to biomedicine. Additionally, in chemistry, the particle model enables the design of new compounds and drugs through molecular engineering, significantly impacting healthcare and pharmaceutical research. The importance of the particle model extends beyond practical applications; it also forms the basis for many theoretical frameworks in physics. Quantum mechanics, for example, relies on the concept of particles like electrons and photons to explain phenomena at the atomic and subatomic level. This theoretical foundation has led to groundbreaking discoveries and technologies, including transistors, lasers, and computer chips. Furthermore, the particle model has facilitated interdisciplinary research. By bridging gaps between physics, chemistry, and biology, it has enabled scientists to study complex systems at multiple scales. For instance, understanding protein structures at the molecular level has been crucial for advances in biotechnology and medicine. This integration of knowledge across disciplines underscores the model's versatility and its role in fostering a holistic understanding of natural phenomena. In educational contexts, the particle model serves as a foundational concept that helps students transition from macroscopic observations to microscopic explanations. It introduces learners to the idea that seemingly continuous substances are actually composed of discrete entities, fostering a deeper appreciation for the intricate structure of matter. This conceptual shift is essential for developing critical thinking and problem-solving skills in science. In conclusion, the particle model of matter is a cornerstone of modern science, offering a robust framework for understanding and explaining a wide range of phenomena. Its importance lies not only in its explanatory power but also in its ability to drive technological innovation, theoretical advancement, and interdisciplinary research. As science continues to evolve, the particle model remains a vital tool for scientists and educators alike, ensuring that our comprehension of the physical world remains both precise and profound.
Components and Structure of the Particle Model
The particle model, a cornerstone of modern physics and chemistry, provides a comprehensive framework for understanding the fundamental components and structure of matter. This model is pivotal in explaining various aspects of physical and chemical phenomena, and it can be dissected into three key areas: Atoms, Molecules, and Subatomic Particles; Chemical Bonding and Interactions; and States of Matter Explained by the Model. At the heart of the particle model lies the concept of atoms, which are the building blocks of matter. These atoms combine to form molecules through chemical bonding, a process that is crucial for understanding the properties and behaviors of substances. The interactions between these molecules dictate the physical states of matter—solid, liquid, and gas—each with distinct characteristics that can be explained by the particle model. Understanding these components and their interactions is essential for grasping the broader implications of the particle model. By delving into the structure and behavior of atoms, molecules, and subatomic particles, we gain a deeper insight into the very fabric of our universe. This foundational knowledge sets the stage for exploring more complex phenomena, such as chemical bonding and the diverse states of matter. Therefore, it is imperative to begin with a thorough examination of **Atoms, Molecules, and Subatomic Particles**, as this forms the basis upon which all other aspects of the particle model are built.
Atoms, Molecules, and Subatomic Particles
The particle model of matter is fundamentally grounded in the understanding of atoms, molecules, and subatomic particles. At the core of this model lies the atom, which is the smallest unit of a chemical element that retains the properties of that element. An atom consists of three primary subatomic particles: protons, neutrons, and electrons. Protons and neutrons reside in the nucleus at the center of the atom, while electrons orbit around it in electron shells or energy levels. The number of protons in an atom defines its chemical identity, determining which element it represents. Molecules are formed when atoms bond together through chemical reactions. These bonds can be ionic, covalent, or metallic, depending on the nature of the interaction between atoms. In ionic bonds, electrons are transferred from one atom to another, resulting in ions that attract each other. Covalent bonds involve the sharing of electron pairs between atoms, typically seen in molecules like water (H₂O) and carbon dioxide (CO₂). Metallic bonds occur in metals where electrons are delocalized across a lattice of atoms. Subatomic particles play a crucial role in understanding atomic structure and behavior. Protons have a positive charge and contribute to the overall charge of the nucleus. Neutrons are neutral and help stabilize the nucleus by balancing the positive charge of protons. Electrons, with their negative charge, occupy specific energy levels or orbitals around the nucleus. The arrangement of electrons in these orbitals is governed by quantum mechanics and determines an atom's chemical reactivity. The particle model also delves into the finer details of subatomic particles such as quarks and leptons, which are part of the Standard Model of particle physics. Quarks combine to form protons and neutrons (collectively known as nucleons), while leptons include electrons and other particles like neutrinos that do not participate in strong nuclear interactions. Understanding these components and their interactions is essential for explaining various phenomena in chemistry and physics. For instance, the periodic table of elements is organized based on the number of protons in an atom's nucleus, reflecting periodic trends in chemical properties. Additionally, molecular structures influence physical properties such as melting points, boiling points, and solubility. In summary, the particle model of matter relies heavily on the intricate relationships between atoms, molecules, and subatomic particles. By grasping how these entities interact at different scales—from atomic nuclei to molecular bonds—the model provides a comprehensive framework for understanding the fundamental nature of matter itself. This foundational knowledge underpins much of modern science and technology, from materials science to biochemistry, highlighting the importance of these microscopic components in our macroscopic world.
Chemical Bonding and Interactions
Chemical bonding and interactions are fundamental aspects of the particle model of matter, as they explain how atoms combine to form molecules and how these molecules interact with each other. At the core of chemical bonding is the concept that atoms seek to achieve a stable electronic configuration, often by gaining, losing, or sharing electrons. This stability is typically achieved when an atom's outermost energy level is fully occupied, akin to the noble gas configuration. **Ionic Bonds** are formed when one or more electrons are transferred from one atom to another, resulting in the formation of ions with opposite charges. These ions then attract each other due to electrostatic forces, forming a strong and rigid bond. For example, sodium (Na) loses an electron to become a positively charged ion (Na⁺), while chlorine (Cl) gains an electron to become a negatively charged ion (Cl⁻). The electrostatic attraction between Na⁺ and Cl⁻ results in the formation of sodium chloride (NaCl), or common table salt. **Covalent Bonds**, on the other hand, involve the sharing of electron pairs between atoms. This type of bonding is prevalent in molecules where atoms share one or more pairs of electrons to achieve a stable electronic configuration. For instance, in a hydrogen molecule (H₂), each hydrogen atom shares its single electron with the other hydrogen atom, forming a single covalent bond. Similarly, in a water molecule (H₂O), oxygen shares its six valence electrons with two hydrogen atoms through two covalent bonds. **Metallic Bonds** occur in metals and involve the delocalization of electrons among a lattice of metal ions. In this type of bonding, electrons are not localized between specific atoms but are free to move throughout the metal lattice. This delocalization of electrons is responsible for the high electrical conductivity and malleability of metals. Beyond these primary types of chemical bonds, there are also weaker interactions that play crucial roles in determining the physical properties of substances. **Van der Waals Forces**, for example, are weak intermolecular forces that arise due to temporary dipoles in molecules. These forces are responsible for the physical state (solid, liquid, or gas) of a substance at room temperature and pressure. **Hydrogen Bonds** are a special type of van der Waals force that occurs between molecules with hydrogen atoms bonded to highly electronegative atoms like oxygen, nitrogen, or fluorine. These bonds are particularly strong and play a significant role in the structure and properties of water and biological molecules. Understanding these various types of chemical bonds and interactions is essential for comprehending the structure and behavior of matter at the molecular level. It explains why certain substances exhibit specific properties such as melting points, boiling points, solubility, and reactivity. Moreover, it underpins many technological and biological processes, from the synthesis of materials to the functioning of biological systems. Thus, chemical bonding and interactions are integral components of the particle model of matter, providing a detailed framework for understanding how atoms combine and interact to form the diverse range of substances we encounter in our world.
States of Matter Explained by the Model
The particle model of matter provides a comprehensive framework for understanding the various states of matter, which are solid, liquid, and gas. This model posits that all matter is composed of tiny particles called atoms or molecules that are in constant motion. The key to understanding the different states lies in the arrangement and movement of these particles. In **solids**, the particles are closely packed and have a fixed position in space. They vibrate slightly due to thermal energy but do not change their relative positions. This rigid structure gives solids their definite shape and volume. For example, in a crystal lattice, atoms or molecules are arranged in a regular, three-dimensional pattern, which contributes to the solid's rigidity and stability. In **liquids**, the particles are still close together but are free to move past one another. This freedom of movement allows liquids to take the shape of their container while maintaining their volume. The particles in liquids have more kinetic energy than those in solids, enabling them to flow and change shape easily. However, they still experience intermolecular forces that keep them from spreading out indefinitely. In **gases**, the particles are widely spaced and are free to move in any direction. The distance between gas molecules is much greater than in solids or liquids, allowing gases to expand and fill any container they occupy. Gas molecules have the highest kinetic energy among the three states, resulting in rapid and random motion. This high energy and large intermolecular distances mean that gases have neither a definite shape nor a definite volume. The transition between these states can be explained by changes in temperature and pressure, which affect the kinetic energy and arrangement of the particles. For instance, heating a solid increases the kinetic energy of its particles, causing them to vibrate more vigorously until they break free from their fixed positions, turning into a liquid (melting). Further heating can cause these liquid particles to gain enough energy to overcome intermolecular forces entirely, turning into a gas (vaporization). The particle model also helps explain other phenomena such as diffusion and Brownian motion. **Diffusion** occurs because gas or liquid particles are in constant random motion, leading them to spread out evenly over time. **Brownian motion**, observed under a microscope, shows how suspended particles move randomly due to collisions with surrounding fluid molecules. In summary, the particle model provides a clear and consistent explanation for the behavior of matter in its various states by focusing on the arrangement and motion of its constituent particles. Understanding these principles allows us to predict how materials will behave under different conditions and is fundamental to fields such as chemistry, physics, and engineering. By recognizing that all matter is composed of tiny moving particles, we can better grasp the underlying mechanisms that govern our physical world.
Applications and Implications of the Particle Model
The particle model, a fundamental concept in physics and chemistry, has far-reaching applications and implications that span multiple disciplines. This model, which describes matter as composed of discrete particles such as atoms and molecules, underpins our understanding of various phenomena. In the realm of **Chemical Reactions and Processes**, the particle model helps explain how substances interact and transform, enabling the prediction and control of chemical reactions. It also sheds light on **Physical Properties of Materials**, allowing us to understand and manipulate the properties of different substances. Furthermore, **Technological Innovations Based on the Model** have led to breakthroughs in fields like materials science, nanotechnology, and energy storage. By grasping the particle model, scientists and engineers can develop new materials, optimize industrial processes, and create innovative technologies. This article will delve into these aspects, starting with the critical role of the particle model in **Chemical Reactions and Processes**.
Chemical Reactions and Processes
Chemical reactions and processes are fundamental to understanding the particle model of matter, as they illustrate how particles interact and transform at the atomic and molecular levels. These reactions involve the breaking and forming of chemical bonds, which are essentially the interactions between electrons and nuclei of atoms. When atoms or molecules collide with sufficient energy, they can rearrange their electron configurations, leading to the formation of new substances. This process is governed by the principles of thermodynamics and kinetics, which dictate the spontaneity and rate of reactions, respectively. In the context of the particle model, chemical reactions can be visualized as the dynamic movement and interaction of particles. For instance, in a combustion reaction, oxygen molecules (O₂) react with a fuel such as methane (CH₄) to produce carbon dioxide (CO₂) and water (H₂O). At the particle level, this involves the collision of oxygen and methane molecules, resulting in the breaking of existing bonds and the formation of new ones. This process releases energy in the form of heat and light, illustrating how chemical reactions can convert one form of energy into another. The implications of these processes are vast and varied. In industrial applications, chemical reactions are harnessed to produce a wide range of materials, from plastics and pharmaceuticals to fuels and fertilizers. For example, the Haber-Bosch process involves the reaction of nitrogen (N₂) with hydrogen (H₂) to produce ammonia (NH₃), a critical component in fertilizer production. This process relies on the precise control of temperature, pressure, and catalysts to optimize the yield of ammonia, demonstrating how understanding particle interactions can lead to efficient industrial processes. Moreover, chemical reactions play a crucial role in biological systems. Metabolic pathways, such as glycolysis and the citric acid cycle, involve a series of complex chemical reactions that convert nutrients into energy. These reactions are catalyzed by enzymes, which are highly specific proteins that lower the activation energy required for the reaction to proceed. Understanding these biochemical processes at the particle level has significant implications for fields like medicine and biotechnology, where insights into metabolic pathways can lead to the development of new treatments for diseases. The particle model also underpins our understanding of environmental processes. For example, the greenhouse effect is driven by chemical reactions involving carbon dioxide and other gases in the Earth's atmosphere. These reactions absorb infrared radiation, trapping heat and contributing to global warming. By studying these processes at the particle level, scientists can better predict climate changes and develop strategies to mitigate them. In conclusion, chemical reactions and processes are integral to the particle model of matter, providing a framework for understanding how particles interact and transform. The applications and implications of these processes span from industrial manufacturing to biological metabolism and environmental science, highlighting the importance of a detailed understanding of particle interactions in various fields. By grasping these fundamental principles, we can develop innovative solutions to complex problems and advance our knowledge of the world around us.
Physical Properties of Materials
The physical properties of materials are fundamentally linked to the particle model of matter, which posits that all substances are composed of tiny, indivisible particles called atoms or molecules. Understanding these properties is crucial for grasping the applications and implications of this model. One key property is **density**, which is determined by the mass and volume of the particles in a material. According to the particle model, density varies because different materials have different atomic or molecular structures, leading to variations in how closely these particles are packed. For instance, metals like lead have high densities due to their tightly packed atomic structures, while gases like helium have low densities because their molecules are widely spaced. Another critical property is **melting and boiling points**, which are influenced by the strength of intermolecular forces between particles. Materials with strong intermolecular forces, such as hydrogen bonds in water or ionic bonds in salts, require more energy to overcome these forces and thus have higher melting and boiling points. Conversely, substances with weaker intermolecular forces, like noble gases with only van der Waals forces, exhibit lower melting and boiling points. This understanding is pivotal in various industrial applications, such as in the design of refrigeration systems or the selection of materials for high-temperature processes. **Thermal conductivity** is another significant property that can be explained through the particle model. Materials with free electrons, such as metals, are good conductors of heat because these electrons can move freely and transfer energy efficiently. In contrast, insulators like wood or plastic have lower thermal conductivity due to their lack of free electrons and weaker intermolecular interactions. This distinction is essential in engineering applications, such as in the development of heat sinks for electronic devices or insulation materials for buildings. The **elasticity** of a material also stems from its particle structure. Elastic materials can deform under stress but return to their original shape once the stress is removed. This behavior is attributed to the ability of particles to move slightly within their lattice structure without breaking the bonds between them. Understanding elasticity is vital in fields like civil engineering, where it influences the design of bridges and buildings that must withstand various loads without permanent deformation. Furthermore, the **optical properties** of materials, such as transparency or opacity, are determined by how particles interact with light. Transparent materials like glass allow light to pass through because their particles do not absorb or scatter light significantly. In contrast, opaque materials like metals reflect light due to the presence of free electrons that interact strongly with light waves. This knowledge is crucial in technologies such as fiber optics and solar panels. In addition, **electrical conductivity** varies widely among materials based on their particle structure. Conductors like copper have high electrical conductivity due to their abundance of free electrons, which can move freely under an electric field. Insulators like rubber have low electrical conductivity because their electrons are tightly bound within atoms or molecules. This distinction is fundamental in electrical engineering, influencing the design of circuits and wiring systems. Lastly, the **magnetic properties** of materials are also rooted in their particle structure. Ferromagnetic materials like iron exhibit strong magnetic behavior due to the alignment of their atomic dipoles, while diamagnetic materials like copper show weak magnetic behavior due to the lack of such alignment. Understanding these properties is essential in applications ranging from magnetic storage devices to medical imaging technologies. In summary, the physical properties of materials—such as density, melting and boiling points, thermal conductivity, elasticity, optical properties, electrical conductivity, and magnetic properties—are all intricately linked to the particle model of matter. By understanding how these properties arise from the interactions and arrangements of particles at the atomic or molecular level, we can better design and utilize materials in various technological and industrial contexts, thereby leveraging the full potential of the particle model in real-world applications.
Technological Innovations Based on the Model
The particle model of matter, which posits that all substances are composed of tiny, indivisible particles called atoms or molecules, has been a cornerstone of scientific understanding for centuries. This fundamental concept has not only shaped our comprehension of the physical world but has also driven numerous technological innovations. One of the most significant implications of the particle model is its role in the development of materials science. By understanding the atomic and molecular structure of materials, scientists and engineers have been able to design and create new materials with specific properties. For instance, nanotechnology relies heavily on manipulating particles at the atomic and molecular level to produce materials with enhanced strength, conductivity, or optical properties. These advancements have led to breakthroughs in fields such as electronics, where nanomaterials are used in high-performance transistors and memory devices, and in medicine, where nanoparticles are employed for targeted drug delivery and imaging. Another critical application of the particle model is in the field of chemistry. The understanding that chemical reactions involve the interaction and rearrangement of atoms has enabled the synthesis of complex molecules with precise properties. This knowledge has been pivotal in the development of pharmaceuticals, where chemists can design drugs that target specific molecular interactions within the body. Additionally, the particle model underpins our understanding of chemical bonding and reactivity, which is essential for the production of polymers, fuels, and other industrial chemicals. In the realm of energy, the particle model has played a crucial role in the development of nuclear power. The concept that atoms can be split (fission) or combined (fusion) to release vast amounts of energy has led to the creation of nuclear reactors and ongoing research into fusion technology. These innovations hold significant promise for addressing global energy needs while reducing carbon emissions. Furthermore, the particle model has profound implications for our understanding of environmental science. By recognizing that pollutants are composed of particles that can interact with and affect the environment at a molecular level, scientists can develop more effective strategies for pollution control and remediation. For example, understanding the particulate nature of air pollutants allows for the design of more efficient air filtration systems and the development of policies aimed at reducing particulate emissions. In computing and information technology, the particle model has influenced the development of semiconductor technology. The precise control over the arrangement of atoms in semiconductor materials is crucial for creating high-performance electronic devices such as microprocessors and memory chips. This level of control is only possible because of our deep understanding of the atomic structure and behavior of these materials. Lastly, the particle model has inspired new approaches in fields like biotechnology and medical research. Understanding the molecular basis of biological processes has enabled the development of gene editing tools like CRISPR/Cas9, which manipulate DNA at the atomic level to treat genetic diseases. Similarly, molecular diagnostics allow for early detection and personalized treatment of diseases by analyzing the molecular signatures of patient samples. In conclusion, the particle model of matter has been a driving force behind a wide range of technological innovations across various disciplines. Its applications span from materials science and chemistry to energy production and environmental science, demonstrating its profound impact on modern technology and society. As our understanding of the particle model continues to evolve, it is likely to inspire even more groundbreaking innovations that shape our future.