What Is The Most Reactive Element
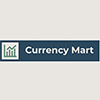
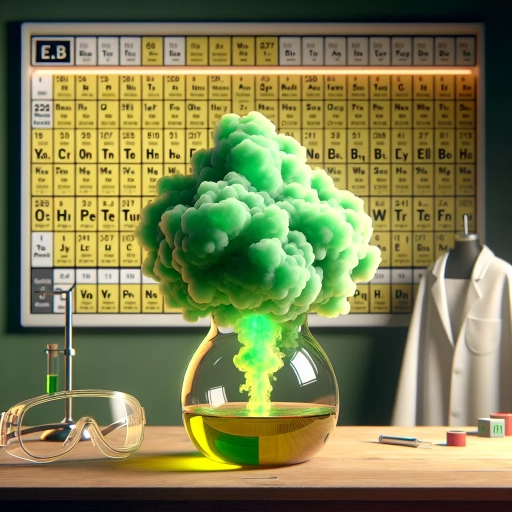
In the vast and intricate world of chemistry, elements exhibit a wide range of reactivity, influencing their interactions and applications. Among these elements, one stands out for its extraordinary reactivity, making it both fascinating and challenging to work with. This article delves into the concept of the most reactive element, exploring its properties, characteristics, and practical uses. We begin by understanding the fundamental principles of reactivity in elements, which sets the stage for identifying and analyzing the most reactive one. Next, we delve into the specific properties and characteristics that make this element so highly reactive. Finally, we discuss its applications and the critical safety considerations that must be taken into account when handling such a potent substance. By grasping these aspects, we can better appreciate the unique role this element plays in various fields. Let's start by understanding reactivity in elements, a crucial foundation for our exploration of the most reactive element.
Understanding Reactivity in Elements
Understanding reactivity in elements is a fundamental concept in chemistry that underpins the interactions and transformations of substances. Reactivity is a measure of how readily an element participates in chemical reactions, forming new compounds or releasing energy. To grasp this complex phenomenon, it is essential to delve into three key aspects: the definition of reactivity, the factors that influence it, and the methods used to measure it. Firstly, defining reactivity sets the groundwork for understanding why certain elements are more prone to reacting than others. This definition will clarify what reactivity entails and how it varies across different elements. Secondly, exploring the factors that influence reactivity will reveal how properties such as electron configuration, atomic size, and electronegativity play crucial roles in determining an element's reactivity. Lastly, understanding how reactivity is measured will provide insights into the quantitative and qualitative methods used to assess an element's ability to participate in chemical reactions. By examining these three facets, we can develop a comprehensive understanding of reactivity in elements. Let us begin by defining what reactivity means in the context of chemistry.
Definition of Reactivity
**Understanding Reactivity in Elements: Definition of Reactivity** Reactivity, a fundamental concept in chemistry, refers to the tendency of an element or compound to undergo chemical reactions. It is a measure of how readily an element can form bonds with other elements or molecules, leading to the formation of new substances. The reactivity of an element is influenced by several key factors, including its electron configuration, particularly the number of electrons in the outermost shell (valence shell), and its position in the periodic table. Elements with a nearly full or nearly empty valence shell are generally more reactive because they tend to gain or lose electrons to achieve a stable noble gas configuration. For instance, alkali metals like sodium and potassium, which have one electron in their outermost shell, are highly reactive as they readily lose this single electron to form a positive ion (cation). Conversely, halogens such as chlorine and fluorine, with seven electrons in their valence shell, are also highly reactive because they easily gain one electron to form a negative ion (anion). The periodic table provides a systematic way to predict reactivity based on an element's position. Elements in Group 1 (alkali metals) and Group 17 (halogens) are typically the most reactive due to their electron configurations. Transition metals, found in the d-block of the periodic table, exhibit variable reactivity depending on their oxidation states and the availability of d-orbitals for bonding. Noble gases, located in Group 18, are the least reactive due to their full valence shells, which make it energetically unfavorable for them to participate in chemical reactions. Reactivity is also influenced by other properties such as electronegativity and ionization energy. Electronegativity measures an atom's ability to attract electrons in a covalent bond; higher electronegativity generally corresponds to higher reactivity for nonmetals. Ionization energy, the energy required to remove an electron from an atom, is lower for highly reactive metals like sodium and potassium, indicating their readiness to lose electrons. Understanding reactivity is crucial for predicting and explaining various chemical phenomena, from combustion reactions involving highly reactive elements like fluorine and oxygen to the synthesis of compounds through controlled reactions. It underpins many industrial processes and technological applications, such as the production of fertilizers from nitrogen-fixing reactions involving highly reactive metals like magnesium and calcium. In summary, reactivity is a complex property that depends on multiple factors including electron configuration, position in the periodic table, electronegativity, and ionization energy. By understanding these principles, chemists can predict how elements will behave in different chemical environments and design reactions that exploit these properties for practical applications. This foundational knowledge is essential for advancing our understanding of chemical processes and developing new materials and technologies.
Factors Influencing Reactivity
Understanding reactivity in elements is fundamentally tied to several key factors that influence how readily an element participates in chemical reactions. One of the primary factors is **electronegativity**, which measures an atom's ability to attract electrons in a covalent bond. Elements with high electronegativity, such as fluorine and oxygen, are highly reactive because they strongly attract electrons, making it easier for them to form bonds with other elements. Conversely, elements with low electronegativity, like cesium and rubidium, are less reactive due to their weaker electron-attracting capabilities. Another crucial factor is **ionization energy**, the energy required to remove an electron from an atom. Elements with low ionization energies, such as those in Group 1 (alkali metals) and Group 2 (alkaline earth metals), are highly reactive because they can easily lose electrons to form positive ions. In contrast, elements with high ionization energies, like noble gases, are less reactive as they require significant energy to remove an electron. **Electron configuration** also plays a pivotal role in determining reactivity. Elements that have nearly full or nearly empty outer energy levels tend to be highly reactive. For instance, alkali metals have one electron in their outermost shell and readily lose this electron to achieve a stable noble gas configuration, making them highly reactive. Similarly, halogens have seven electrons in their outermost shell and are highly reactive as they seek to gain one electron to achieve a stable noble gas configuration. The **size of the atom** is another influential factor. Smaller atoms have higher electron density and stronger nuclear attraction, which can make them less reactive. However, smaller atoms also have higher ionization energies but lower electron affinities, which can sometimes counterbalance their reactivity. Larger atoms, particularly those in the lower periods of the periodic table, tend to be more reactive due to their lower ionization energies and higher electron affinities. **Valence electrons** and their arrangement significantly impact reactivity. Elements with multiple valence electrons can form multiple bonds or participate in complex reactions more easily than those with fewer valence electrons. For example, carbon has four valence electrons and can form a wide variety of compounds due to its ability to form single, double, and triple bonds. Lastly, **the presence of d or f orbitals** in transition metals and actinides/lanthanides respectively introduces additional complexity to their reactivity. These elements can exhibit variable valency and form ions with different charges, making them versatile in chemical reactions. In summary, understanding the interplay of these factors—electronegativity, ionization energy, electron configuration, atomic size, valence electrons, and the presence of d or f orbitals—provides a comprehensive insight into why certain elements are more reactive than others. These factors collectively determine an element's propensity to engage in chemical reactions and form compounds, making them essential for predicting and explaining reactivity trends across the periodic table.
Measurement of Reactivity
**Measurement of Reactivity** Understanding the reactivity of elements is crucial in chemistry, and measuring this property involves several key methods. Reactivity, essentially, is a measure of how readily an element participates in chemical reactions. One of the primary ways to quantify reactivity is through the use of reactivity series or activity series, which rank metals based on their ability to lose electrons and form ions. For metals, this series is often determined by observing their ability to displace other metals from solutions of their salts. For instance, if a metal can displace another metal from its salt solution, it is considered more reactive. Another critical method involves measuring the standard electrode potential (E°) of an element. This value indicates the tendency of an element to lose or gain electrons when it is part of an electrochemical cell. Elements with highly negative E° values are highly reactive because they readily lose electrons to form ions. Conversely, elements with positive E° values are less reactive as they tend to gain electrons. The ionization energy and electron affinity of an element also provide insights into its reactivity. Ionization energy, the energy required to remove an electron from an atom, is lower for highly reactive elements like alkali metals, indicating their ease in losing electrons. Electron affinity, which is the energy released when an electron is added to an atom, is particularly relevant for nonmetals; highly reactive nonmetals such as fluorine have high electron affinities, reflecting their strong tendency to gain electrons. In addition to these quantitative measures, qualitative observations such as the speed and vigor of reactions can also indicate an element's reactivity. For example, highly reactive elements like potassium and sodium react violently with water, producing hydrogen gas and an alkaline solution, while less reactive metals like copper and silver do not react with water under normal conditions. Furthermore, the position of an element in the periodic table offers valuable clues about its reactivity. Elements in Group 1 (alkali metals) and Group 17 (halogens) are generally highly reactive due to their tendency to lose one electron or gain one electron, respectively, to achieve a stable noble gas configuration. In contrast, elements in the noble gas group (Group 18) are unreactive because they already possess a full outer energy level. In summary, measuring the reactivity of elements involves a combination of quantitative and qualitative approaches. By using reactivity series, standard electrode potentials, ionization energies, electron affinities, and observing reaction behaviors, chemists can comprehensively understand and predict how different elements will react under various conditions. This understanding is fundamental for predicting chemical behavior and designing experiments or industrial processes that involve these elements.
The Most Reactive Element: Properties and Characteristics
The most reactive element, known for its extraordinary ability to form bonds and undergo chemical reactions, is a subject of great interest in chemistry. Understanding its properties and characteristics is crucial for grasping its behavior in various chemical environments. This article delves into the key aspects that define this element's reactivity, starting with its electron configuration and valence electrons. We will explore how the arrangement of electrons in the atom influences its chemical behavior. Additionally, we will examine the ionization energy and electron affinity, which are critical in determining how easily the element loses or gains electrons. Finally, we will discuss its chemical behavior in different environments, highlighting how these properties manifest in real-world scenarios. By understanding these three fundamental aspects—electron configuration and valence electrons, ionization energy and electron affinity, and chemical behavior in different environments—we can gain a comprehensive insight into what makes this element so reactive. Let us begin by examining the electron configuration and valence electrons, which form the foundation of its reactivity.
Electron Configuration and Valence Electrons
Electron configuration and valence electrons are fundamental concepts in understanding the reactivity of elements, particularly when identifying the most reactive ones. Electron configuration refers to the arrangement of electrons in an atom, which is crucial for determining its chemical properties. This arrangement is based on the Aufbau principle, the Pauli exclusion principle, and Hund's rule, which dictate how electrons occupy orbitals around the nucleus. The outermost energy level of an atom, known as the valence shell, contains valence electrons. These electrons are pivotal in forming chemical bonds and thus play a significant role in an element's reactivity. Valence electrons are those found in the highest principal energy level of an atom and are involved in chemical bonding. The number of valence electrons an atom has determines its position in the periodic table and its chemical behavior. For instance, elements in Group 1 (alkali metals) have one valence electron, making them highly reactive as they readily lose this single electron to achieve a stable noble gas configuration. Conversely, elements in Group 17 (halogens) have seven valence electrons and are also highly reactive because they need only one more electron to complete their outer shell. The reactivity of an element is often linked to its tendency to gain or lose electrons to achieve a stable electronic configuration. Elements with a full outer shell (noble gases) are generally unreactive due to their stable electron configuration. In contrast, elements with nearly full or nearly empty outer shells are more reactive because they can easily gain or lose electrons to achieve stability. For example, cesium (Cs), an alkali metal with one valence electron, is highly reactive because it readily loses this electron to form a positive ion (Cs⁺), while fluorine (F), a halogen with seven valence electrons, is highly reactive because it readily gains an electron to form a negative ion (F⁻). Understanding electron configuration and valence electrons is essential for predicting the chemical behavior of elements. This knowledge helps in identifying which elements are likely to be highly reactive and why certain elements form specific types of bonds. For instance, the high reactivity of alkali metals like cesium and rubidium can be attributed to their single valence electron, which they can easily lose to form ions. Similarly, the high reactivity of halogens like fluorine and chlorine is due to their need for just one additional electron to complete their outer shell. In the context of identifying the most reactive element, understanding electron configuration and valence electrons provides a clear framework. The most reactive elements typically have either one or seven valence electrons, making them prone to losing or gaining electrons quickly. This reactivity is a key characteristic that distinguishes these elements from others and explains their vigorous participation in chemical reactions. By analyzing the electron configuration and valence electrons of various elements, scientists can predict their reactivity and understand why certain elements exhibit specific chemical properties, making this knowledge indispensable in chemistry and materials science.
Ionization Energy and Electron Affinity
Ionization energy and electron affinity are two fundamental concepts in chemistry that play crucial roles in understanding the reactivity of elements. **Ionization energy** is the amount of energy required to remove an electron from a neutral atom in its ground state, typically measured in electronvolts (eV). This energy barrier is a key indicator of an element's tendency to lose electrons and form cations. Elements with low ionization energies, such as those in Group 1 (alkali metals) and Group 2 (alkaline earth metals), are highly reactive because they can easily release electrons to achieve a stable noble gas configuration. For instance, cesium (Cs) has one of the lowest ionization energies among all elements, making it highly reactive and prone to losing its single valence electron. On the other hand, **electron affinity** is the energy change when an electron is added to a neutral atom in its ground state. It is a measure of how readily an atom can gain an electron to form an anion. Unlike ionization energy, electron affinity can be either positive or negative; a negative value indicates that energy is released when an electron is added, suggesting that the atom has a strong tendency to gain electrons. Elements with high negative electron affinities, such as those in Group 17 (halogens), are highly reactive because they readily accept electrons to complete their outer shell and achieve a stable noble gas configuration. Chlorine (Cl), for example, has a high negative electron affinity, which explains its strong tendency to form chloride ions (Cl^-). The interplay between ionization energy and electron affinity is critical in determining the overall reactivity of an element. For instance, fluorine (F) has both a low ionization energy and a high negative electron affinity, making it one of the most reactive elements known. Its ability to easily lose and gain electrons facilitates its participation in numerous chemical reactions. In contrast, noble gases like neon (Ne) have high ionization energies and low (or even positive) electron affinities, rendering them chemically inert due to their stable full outer shell configuration. Understanding these properties is essential for predicting how elements will behave in various chemical environments. For example, in the context of the most reactive element, cesium's low ionization energy and fluorine's high negative electron affinity make them stand out as exceptionally reactive. These characteristics underpin their participation in vigorous reactions and their ability to form compounds with other elements readily. Thus, grasping ionization energy and electron affinity provides a foundational insight into why certain elements exhibit extreme reactivity and how they interact with other substances at the atomic level.
Chemical Behavior in Different Environments
Chemical behavior in different environments is a critical aspect of understanding the reactivity of elements, particularly when discussing the most reactive element. The reactivity of an element is influenced by its electronic configuration, which dictates how readily it gains or loses electrons to form compounds. In various environments, such as aqueous solutions, gaseous phases, and solid states, the chemical behavior of an element can significantly vary due to changes in temperature, pressure, and the presence of other substances. For instance, in aqueous solutions, highly reactive elements like cesium and francium exhibit vigorous reactions with water, releasing hydrogen gas and forming alkaline solutions. This is because these alkali metals have a single electron in their outermost shell, which they readily lose to achieve a stable noble gas configuration. In contrast, in gaseous phases at high temperatures, these metals can react with gases such as oxygen and nitrogen to form oxides and nitrides. The reactivity in gaseous environments is often enhanced by the increased kinetic energy of the atoms or molecules, facilitating more frequent and energetic collisions that lead to chemical reactions. In solid states, the reactivity can be influenced by the crystal structure and the availability of surface sites for reaction. For example, highly reactive metals may react with atmospheric oxygen to form a protective oxide layer when exposed to air. This oxide layer can sometimes passivate the metal's surface, reducing its reactivity over time. However, in environments where this oxide layer is disrupted or absent, such as in the presence of moisture or certain chemicals, the metal's reactivity can be significantly enhanced. The presence of catalysts or other substances can also alter an element's chemical behavior. For instance, certain transition metals can catalyze reactions by providing alternative pathways with lower activation energies, thereby increasing the rate of reaction without being consumed in the process. Additionally, solvents and ligands can coordinate with reactive elements to form complexes that either stabilize or destabilize their electronic configurations, thus modulating their reactivity. Understanding these environmental factors is crucial for predicting and controlling the chemical behavior of highly reactive elements. This knowledge is essential in various applications, from chemical synthesis and materials science to energy storage and environmental remediation. By recognizing how different environments influence an element's reactivity, scientists can design safer handling protocols, optimize reaction conditions, and develop new technologies that leverage these properties effectively. In summary, the chemical behavior of elements, especially the most reactive ones, is highly dependent on their surroundings. Whether in aqueous solutions, gaseous phases, or solid states, the interplay between environmental conditions and electronic configurations determines how these elements interact with other substances. This nuanced understanding is vital for harnessing their potential while ensuring safety and efficiency in various scientific and industrial contexts.
Applications and Safety Considerations of the Most Reactive Element
The most reactive element, known for its intense chemical activity, presents a complex landscape of applications and safety considerations. This element, while highly valuable in various industrial processes, demands stringent safety protocols to mitigate its hazardous nature. In this article, we delve into the multifaceted aspects of this element's use and management. We begin by exploring its **Industrial Uses and Applications**, highlighting how it is leveraged in manufacturing, energy production, and other sectors. This discussion sets the stage for understanding the necessity of **Safety Protocols and Handling Precautions**, which are crucial for preventing accidents and ensuring worker safety. Additionally, we examine the **Environmental Impact and Regulations** surrounding its use, emphasizing the importance of responsible handling to protect ecosystems and comply with regulatory standards. By understanding these interconnected facets, we can harness the benefits of this reactive element while minimizing its risks. Let us first explore how this element is utilized in industrial settings, where its reactivity is both a boon and a challenge.
Industrial Uses and Applications
The most reactive element, typically considered to be fluorine due to its high electronegativity and reactivity, has a multitude of industrial uses and applications that leverage its unique properties. In the chemical industry, fluorine is crucial for the production of fluoropolymers such as Teflon (polytetrafluoroethylene) and fluorinated ethylene propylene (FEP), which are renowned for their non-stick surfaces, high temperature resistance, and chemical inertness. These materials are widely used in cookware, electrical insulation, and medical devices. Additionally, fluorine is a key component in the synthesis of refrigerants like chlorofluorocarbons (CFCs) and hydrofluorocarbons (HFCs), although the use of CFCs has been significantly curtailed due to their contribution to ozone depletion. In the pharmaceutical sector, fluorine plays a pivotal role in drug development. Fluorinated compounds are often used to enhance the bioavailability and stability of drugs, making them more effective and longer-lasting. For instance, many modern medications, including certain antidepressants and antiviral drugs, contain fluorine atoms that improve their pharmacokinetic profiles. The semiconductor industry also relies heavily on fluorine compounds, particularly in the etching processes necessary for fabricating microelectronic devices. Hydrofluoric acid, a highly reactive compound containing fluorine, is used to etch silicon wafers and create the intricate patterns required for integrated circuits. Furthermore, fluorine is essential in the production of nuclear fuels. Uranium hexafluoride (UF6) is a critical intermediate in the enrichment of uranium for nuclear reactors and weapons. This compound's volatility allows it to be efficiently separated using gas centrifuges or gaseous diffusion methods. In dental care, fluoride compounds are widely recognized for their ability to prevent tooth decay and strengthen tooth enamel. Fluoridated water and toothpaste have significantly reduced the incidence of dental caries worldwide. Despite these numerous applications, the handling of fluorine requires stringent safety measures due to its extreme reactivity and toxicity. Industrial processes involving fluorine must be conducted in controlled environments with appropriate ventilation and protective gear to prevent exposure. The storage and transportation of fluorine-containing compounds also necessitate specialized containers and safety protocols to mitigate risks associated with leaks or spills. In summary, the industrial uses of fluorine are diverse and critical across various sectors, from chemical manufacturing and pharmaceuticals to semiconductor fabrication and nuclear fuel production. While its reactivity presents significant challenges, careful management and adherence to safety protocols ensure that these applications can be harnessed effectively without compromising worker safety or environmental integrity.
Safety Protocols and Handling Precautions
When dealing with the most reactive elements, such as fluorine or cesium, stringent safety protocols and handling precautions are paramount to prevent accidents and ensure the well-being of individuals involved. These elements are highly volatile and can react violently with air, water, and other substances, making their handling a delicate task. First and foremost, personal protective equipment (PPE) is essential. This includes wearing full-body suits, gloves, goggles, and face shields to protect against splashes and spills. The use of self-contained breathing apparatuses (SCBAs) or supplied air respirators is also crucial to avoid inhalation of toxic fumes. Additionally, working in well-ventilated areas or fume hoods can help mitigate the risks associated with airborne contaminants. The storage of these reactive elements requires specialized containers that are designed to withstand their reactivity. For instance, fluorine is often stored in passivated nickel or Monel containers to prevent violent reactions. These containers must be kept in cool, dry places away from any potential ignition sources or incompatible substances. Handling procedures should be meticulously planned and executed. When transferring these elements, it is advisable to use tongs or other tools to minimize direct contact. Any equipment used must be thoroughly cleaned and dried to prevent accidental ignition or explosion. It is also important to have emergency response plans in place, including access to fire extinguishers rated for chemical fires and emergency showers or eye wash stations. Training is another critical aspect of safety protocols. Personnel handling these elements must undergo comprehensive training that includes understanding the chemical properties of the substances, recognizing potential hazards, and knowing how to respond in case of an emergency. Regular drills and simulations can help reinforce this knowledge and ensure preparedness. Furthermore, regulatory compliance is vital. Adhering to guidelines set by organizations such as the Occupational Safety and Health Administration (OSHA) and the National Institute for Occupational Safety and Health (NIOSH) ensures that all safety standards are met. This includes maintaining accurate records of handling procedures, storage conditions, and any incidents that may occur. In summary, the safe handling of the most reactive elements demands a multifaceted approach that includes proper PPE, specialized storage and handling techniques, thorough training, and strict adherence to regulatory guidelines. By implementing these safety protocols and precautions diligently, we can significantly reduce the risks associated with working with these highly reactive substances, thereby protecting both individuals and the environment from potential harm.
Environmental Impact and Regulations
The environmental impact and regulations surrounding the most reactive element, typically considered to be fluorine or cesium depending on the context, are critical considerations in both industrial applications and scientific research. Fluorine, for instance, is highly reactive and forms compounds with almost all elements, including noble gases. Its reactivity makes it a valuable component in various industries such as semiconductor manufacturing, pharmaceuticals, and the production of fluoropolymers like Teflon. However, this reactivity also poses significant environmental risks. Fluorine compounds can be harmful to ecosystems if released into the atmosphere or water bodies. For example, fluorinated gases (F-gases) are potent greenhouse gases that contribute to climate change, while fluorides can accumulate in soil and water, leading to environmental pollution and health issues. To mitigate these risks, stringent regulations have been implemented globally. The Montreal Protocol, an international treaty signed in 1987, aims to phase out substances that deplete the ozone layer, including certain fluorinated compounds. Additionally, the Kyoto Protocol and subsequent agreements like the Paris Agreement have targeted reductions in greenhouse gas emissions, including those from F-gases. In the United States, the Environmental Protection Agency (EPA) regulates fluorine-containing substances under various laws such as the Clean Air Act and the Toxic Substances Control Act (TSCA). These regulations mandate strict handling, storage, and disposal practices for fluorine and its compounds to prevent environmental contamination. Moreover, companies involved in the production and use of fluorine must adhere to best management practices (BMPs) that include leak detection and repair programs, proper waste management, and employee training on safe handling procedures. Research institutions also follow rigorous safety protocols when working with highly reactive elements like fluorine or cesium. These protocols often include the use of specialized equipment such as fume hoods, personal protective equipment (PPE), and emergency response plans in case of accidents. In conclusion, while the most reactive elements offer significant benefits across various industries due to their unique properties, their environmental impact necessitates robust regulatory frameworks and stringent safety measures. Compliance with these regulations not only protects the environment but also ensures the health and safety of workers and communities surrounding industrial facilities. As research continues to explore new applications for these elements, it is imperative that environmental sustainability remains a paramount consideration to balance technological advancement with ecological responsibility.