What Is Frequency Measured In
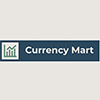
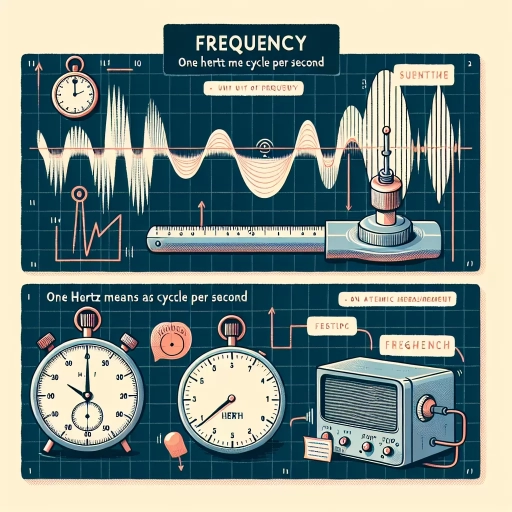
Frequency, a fundamental concept in physics and engineering, is a measure of the number of occurrences or cycles of a repeating event per unit of time. It is a crucial parameter in various fields, including physics, electronics, and telecommunications. Understanding frequency is essential for grasping how different phenomena operate and interact. This article delves into the multifaceted nature of frequency, starting with **Understanding Frequency Basics**, where we explore the definitions, units, and fundamental principles that govern frequency. We then move on to **Measurement Techniques and Tools**, discussing the methods and instruments used to accurately measure frequency in different contexts. Finally, we examine **Practical Uses Across Disciplines**, highlighting how frequency plays a pivotal role in fields such as music, medicine, and technology. By understanding these aspects, readers will gain a comprehensive insight into the significance and applications of frequency. Let's begin by laying the groundwork with **Understanding Frequency Basics**.
Understanding Frequency Basics
Understanding frequency basics is a fundamental concept in various fields, including physics, engineering, and telecommunications. To grasp the essence of frequency, it is crucial to delve into three key aspects: the definition of frequency, the units of measurement, and its common applications. Firstly, understanding what frequency is at its core sets the stage for further exploration. Frequency is defined as the number of cycles or oscillations per second of a wave or signal. This foundational knowledge is essential for comprehending how frequencies operate and interact within different systems. Secondly, recognizing the units of measurement for frequency is vital. The most common unit is the Hertz (Hz), which quantifies the number of cycles per second. Other units, such as kilohertz (kHz) and megahertz (MHz), are also used depending on the context and scale. Lastly, exploring the common applications of frequency reveals its widespread importance. From radio waves and sound waves to medical imaging and data transmission, frequency plays a critical role in many technologies that shape our daily lives. By starting with a clear definition of frequency, we can build a robust understanding that extends into its measurement and practical uses. Therefore, let us begin by examining the definition of frequency in greater detail.
Definition of Frequency
**Understanding Frequency Basics** ### Definition of Frequency Frequency, a fundamental concept in physics and engineering, is defined as the number of occurrences or cycles of a repeating event per unit of time. It is a measure of how often something happens within a given timeframe. Mathematically, frequency is expressed as the reciprocal of the period, which is the time taken for one complete cycle. For instance, if a wave completes one cycle in 0.5 seconds, its frequency would be 2 cycles per second (or 2 Hz). This concept is crucial in various fields such as acoustics, electromagnetism, and signal processing. In practical terms, frequency can be observed in everyday phenomena. For example, the frequency of a musical note determines its pitch; higher frequencies correspond to higher pitches. Similarly, in electronics, the frequency of an alternating current (AC) determines how often the current changes direction. The standard household AC frequency is typically 50 or 60 Hz, depending on the region. The unit of measurement for frequency is the Hertz (Hz), named after Heinrich Hertz, who demonstrated the existence of electromagnetic waves. One Hertz equals one cycle per second. Other units like kilohertz (kHz), megahertz (MHz), and gigahertz (GHz) are used for higher frequencies. Understanding frequency is essential for designing and analyzing systems that involve periodic phenomena, such as radio communication systems, medical imaging devices, and musical instruments. Frequency also plays a critical role in data analysis and signal processing. In these contexts, it helps in identifying patterns and trends within datasets. For instance, in audio processing, frequency analysis can help in noise reduction and sound enhancement by isolating specific frequency bands. Moreover, the concept of frequency extends beyond physical phenomena to statistical analysis. In statistics, frequency refers to the number of times an event occurs within a dataset. This is often visualized through frequency distributions or histograms, which provide insights into the distribution of data points. In summary, frequency is a versatile and essential concept that underpins many scientific and engineering disciplines. Its precise measurement and understanding are vital for both theoretical and practical applications across various fields, making it a cornerstone of modern technology and scientific inquiry.
Units of Measurement
Understanding the units of measurement is crucial when delving into frequency basics, as it provides a clear framework for quantifying and comparing different frequencies. Frequency, which is the number of cycles or oscillations per second, is typically measured in Hertz (Hz). This unit honors Heinrich Hertz, a German physicist who demonstrated the existence of electromagnetic waves. The Hertz is defined as one cycle per second, making it a fundamental unit in the International System of Units (SI). In practical applications, frequencies can span a wide range, necessitating the use of various prefixes to denote different orders of magnitude. For instance, kilohertz (kHz) represents 1,000 Hz, megahertz (MHz) represents 1 million Hz, and gigahertz (GHz) represents 1 billion Hz. These prefixes are essential for describing frequencies in different contexts, such as radio waves (often in kHz or MHz), computer processor speeds (often in GHz), and even the frequencies of light (which can be in the terahertz range). Beyond these common units, there are other specialized units that may be encountered in specific fields. For example, in audio engineering, frequencies might be discussed in terms of octaves or semitones to describe musical intervals. In physics and engineering, angular frequency (measured in radians per second) is sometimes used to describe rotational motion or oscillations. The precision and consistency of these measurement units are vital for scientific and technological advancements. For instance, in telecommunications, precise frequency measurements ensure that signals are transmitted and received correctly without interference. Similarly, in medical imaging techniques like MRI (Magnetic Resonance Imaging), accurate frequency measurements are critical for producing clear images. Moreover, understanding the units of measurement allows for meaningful comparisons across different domains. For example, knowing that a typical FM radio station broadcasts at around 100 MHz helps in understanding why it operates at a much lower frequency than a microwave oven, which operates at about 2.45 GHz. In conclusion, mastering the units of measurement for frequency is essential for grasping its basics and applications. Whether you are dealing with radio waves, computer hardware, or medical imaging, these units provide a common language that facilitates communication and ensures accuracy in various fields. By recognizing the significance of Hertz and its derivatives, one can better comprehend how frequency plays a pivotal role in modern technology and science.
Common Applications
Understanding frequency basics is crucial for grasping its myriad applications across various fields. Frequency, measured in Hertz (Hz), represents the number of cycles or oscillations per second of a wave or signal. This fundamental concept underpins numerous technologies and phenomena that shape our daily lives. In telecommunications, frequency is essential for radio and television broadcasting, where different frequencies are allocated to different channels to prevent interference. For instance, AM radio operates on medium frequencies (MF) between 535 and 1605 kHz, while FM radio uses very high frequencies (VHF) ranging from 88 to 108 MHz. Similarly, cellular networks rely on specific frequency bands to enable mobile communication, with 4G networks using frequencies such as 700 MHz and 5G networks leveraging higher bands like millimeter wave frequencies above 24 GHz. In medicine, frequency plays a critical role in diagnostic and therapeutic technologies. Ultrasound imaging, for example, uses high-frequency sound waves (typically between 2 and 10 MHz) to create detailed images of internal organs. Magnetic Resonance Imaging (MRI) also relies on the principles of frequency, utilizing radiofrequency pulses to align hydrogen nuclei in the body, which then emit signals that are used to construct detailed images. Additionally, medical treatments like radiofrequency ablation use controlled heat generated by high-frequency electrical currents to destroy abnormal tissues. Frequency is equally important in music and audio engineering. Musical instruments produce sound waves at specific frequencies, with the standard concert pitch A set at 440 Hz. Audio equipment such as speakers and microphones are designed to handle a range of frequencies, typically spanning from 20 Hz (the lowest audible frequency) to 20 kHz (the highest audible frequency). Equalizers in audio systems adjust the amplitude of different frequency bands to enhance or reduce specific tones, improving overall sound quality. In power systems, frequency stability is vital for maintaining grid reliability. Electrical power grids operate at a standard frequency of 50 or 60 Hz, depending on the region. Deviations from these frequencies can indicate issues such as overloading or underloading of the grid, necessitating immediate corrective actions to prevent blackouts or equipment damage. Furthermore, frequency analysis is a cornerstone of data analysis and signal processing. Techniques like Fast Fourier Transform (FFT) decompose complex signals into their constituent frequencies, allowing for the identification of patterns and anomalies that might not be apparent in time-domain data. This is particularly useful in fields such as seismology, where analyzing the frequency content of seismic waves helps scientists understand the structure of the Earth's interior. In conclusion, understanding frequency basics opens up a wide array of applications across telecommunications, medicine, music, power systems, and data analysis. Recognizing how different frequencies are utilized and managed in these contexts not only enhances our appreciation for the underlying technology but also underscores the importance of precise frequency control in ensuring the reliability and efficiency of modern systems. By grasping these principles, we can better appreciate the intricate role that frequency plays in shaping our technological landscape.
Measurement Techniques and Tools
In the realm of measurement techniques and tools, precision and accuracy are paramount. Modern engineering and scientific endeavors rely heavily on a variety of sophisticated instruments to gather and analyze data. This article delves into three critical categories of measurement tools that have revolutionized the field: Electronic Frequency Counters, Spectrum Analyzers, and Software-Based Measurement Tools. Each of these tools plays a unique role in ensuring the integrity and reliability of measurements across diverse applications. Electronic Frequency Counters are essential for accurately measuring the frequency of signals, a fundamental aspect in telecommunications and electronics. Spectrum Analyzers, on the other hand, provide comprehensive insights into signal composition and behavior over a wide range of frequencies. Meanwhile, Software-Based Measurement Tools offer flexibility and adaptability, enabling real-time data analysis and customization. By exploring these three types of measurement tools, we can gain a deeper understanding of how they collectively enhance our ability to measure and interpret data with precision. Let us begin by examining the role of Electronic Frequency Counters in modern measurement practices.
Electronic Frequency Counters
**Electronic Frequency Counters** In the realm of measurement techniques and tools, electronic frequency counters stand as indispensable instruments for accurately determining the frequency of signals. These devices are crucial in various fields such as telecommunications, electronics engineering, and scientific research. An electronic frequency counter measures the number of cycles or oscillations of a signal within a specified time period, typically one second, to provide a precise frequency reading. The operation of an electronic frequency counter involves several key components. The input signal is first processed by a preamplifier to enhance its amplitude and improve signal-to-noise ratio. This amplified signal is then fed into a gate circuit that opens and closes at precise intervals, allowing the signal to pass through during these intervals. The gate is usually controlled by a crystal oscillator, which provides a highly stable reference frequency. The number of signal cycles passing through the gate during each interval is counted by digital counters, and this count is displayed on an LCD or LED screen. Modern electronic frequency counters offer a range of features that enhance their functionality and accuracy. High-resolution counters can measure frequencies with precision down to fractions of a hertz, while others may include additional capabilities such as period measurement, pulse counting, and even phase noise analysis. Some advanced models also incorporate automatic gain control (AGC) to adjust the input sensitivity dynamically, ensuring optimal performance across a wide range of input signal levels. The versatility of electronic frequency counters makes them essential tools in various applications. In telecommunications, they are used to verify the frequency stability of transmitters and receivers. In electronics manufacturing, they help in the quality control of oscillators and other frequency-dependent components. In scientific research, they are invaluable for studying phenomena that involve periodic signals, such as in physics and engineering experiments. Moreover, advancements in technology have led to the development of handheld and portable frequency counters, making them accessible for field measurements. These compact devices often include battery operation and rugged designs, allowing engineers and technicians to perform on-site measurements with ease. In conclusion, electronic frequency counters are sophisticated measurement tools that play a vital role in ensuring the accuracy and reliability of frequency measurements across diverse industries. Their precision, versatility, and ease of use make them an indispensable asset in any setting where frequency measurement is critical. As technology continues to evolve, it is likely that electronic frequency counters will become even more sophisticated, offering higher precision and additional functionalities to meet the demands of an increasingly complex technological landscape.
Spectrum Analyzers
**Spectrum Analyzers** Spectrum analyzers are sophisticated measurement tools that play a crucial role in the analysis and understanding of signal frequencies across various domains, including telecommunications, engineering, and scientific research. These devices are designed to measure the magnitude of an input signal versus frequency within a given frequency range, providing a visual representation of the signal's spectral content. This capability is essential for diagnosing issues, optimizing performance, and ensuring compliance with regulatory standards. At the heart of a spectrum analyzer lies its ability to decompose complex signals into their constituent frequencies. This is achieved through techniques such as Fast Fourier Transform (FFT) or swept-tuned methods, which allow the analyzer to scan the frequency spectrum and display the power density of the signal at each frequency point. The resulting spectrum display can reveal critical information about signal characteristics, including amplitude, frequency, and modulation. One of the key applications of spectrum analyzers is in the field of telecommunications. Here, they are used to monitor and troubleshoot wireless communication systems, ensuring that signals adhere to specified frequency bands and do not interfere with other transmissions. In engineering, spectrum analyzers help in the design and testing of electronic circuits by identifying unwanted harmonics or spurious emissions that could affect system performance. In addition to their technical applications, spectrum analyzers are also invaluable in scientific research. For instance, in astronomy, these tools help analyze the spectral signatures of celestial objects, providing insights into their composition and behavior. Similarly, in medical research, spectrum analyzers can be used to study the electromagnetic properties of biological tissues. The modern spectrum analyzer offers a range of advanced features that enhance its functionality. These include high-resolution displays, advanced triggering options, and the ability to perform real-time analysis. Some models also support vector signal analysis, which allows for the detailed examination of both amplitude and phase information in signals. Furthermore, many contemporary spectrum analyzers are equipped with software capabilities that enable data logging, automated testing, and integration with other measurement tools. In summary, spectrum analyzers are indispensable tools in the arsenal of measurement techniques and tools. Their ability to provide detailed spectral information makes them crucial for diagnosing issues, optimizing system performance, and advancing scientific knowledge across diverse fields. As technology continues to evolve, the role of spectrum analyzers will only become more significant, enabling precise and comprehensive analysis of signal frequencies in an increasingly complex world.
Software-Based Measurement Tools
**Software-Based Measurement Tools** In the realm of measurement techniques, software-based measurement tools have revolutionized the way data is collected, analyzed, and interpreted. These tools leverage advanced algorithms and sophisticated software architectures to provide precise and reliable measurements across various domains. Unlike traditional hardware-based tools, software-based measurement tools offer flexibility, scalability, and cost-effectiveness, making them indispensable in modern scientific research, engineering, and industrial applications. One of the key advantages of software-based measurement tools is their ability to integrate with a wide range of hardware devices. For instance, in the field of frequency measurement, software-defined instruments can interface with oscilloscopes, spectrum analyzers, and signal generators to capture and analyze signals with high accuracy. These tools often include user-friendly interfaces that allow users to configure settings, visualize data in real-time, and perform complex analyses without the need for extensive technical expertise. Moreover, software-based measurement tools are highly adaptable and can be easily updated to accommodate new measurement protocols or standards. This adaptability is particularly beneficial in fields like telecommunications and electronics, where standards and technologies evolve rapidly. For example, software-defined radio (SDR) platforms can be reconfigured to support different communication protocols simply by updating the software, eliminating the need for hardware changes. Another significant benefit of these tools is their capacity for automation. Automated measurement scripts can be written to perform repetitive tasks, ensuring consistency and reducing human error. This is particularly useful in high-volume testing environments such as manufacturing quality control, where precise and consistent measurements are crucial for ensuring product reliability. Additionally, software-based measurement tools facilitate data sharing and collaboration. Measurement data can be easily exported in various formats and shared across different platforms, enabling seamless collaboration among researchers and engineers. This feature is especially valuable in interdisciplinary projects where data needs to be analyzed from multiple perspectives. From a cost perspective, software-based measurement tools often provide a more economical solution compared to traditional hardware-based alternatives. The initial investment in hardware can be minimized as the same hardware can be repurposed with different software configurations. Furthermore, the maintenance and upgrade costs are significantly lower since updates are typically done through software patches rather than hardware replacements. In conclusion, software-based measurement tools have transformed the landscape of measurement techniques by offering unparalleled flexibility, precision, and cost-effectiveness. Their ability to integrate with various hardware devices, adapt to changing standards, automate repetitive tasks, facilitate data sharing, and reduce costs makes them an essential component in modern measurement practices. As technology continues to evolve, the role of these tools will only become more pivotal in ensuring accurate and reliable measurements across diverse fields.
Practical Uses Across Disciplines
The practical uses of advanced technologies and methodologies span a wide array of disciplines, each contributing uniquely to our understanding and innovation. In the realm of **Physics and Engineering**, these applications are pivotal in developing cutting-edge solutions for energy, transportation, and materials science. For instance, quantum mechanics informs the design of more efficient solar cells and advanced materials, while engineering principles guide the construction of sustainable infrastructure. Beyond the physical sciences, **Music and Acoustics** benefit significantly from technological advancements. The study of sound waves and resonance has led to the development of superior audio equipment and concert halls with optimal acoustic properties. This intersection of technology and art enhances our auditory experiences and pushes the boundaries of musical expression. In **Telecommunications and Signal Processing**, these technologies enable faster, more reliable communication networks. Signal processing algorithms improve data transmission rates and quality, ensuring seamless communication across the globe. These advancements are crucial for modern communication systems, from mobile networks to satellite communications. Understanding these diverse applications underscores the interconnected nature of scientific and technological progress. By exploring how these disciplines intersect, we can appreciate the broader impact of innovation. Let us delve into the specifics of how **Physics and Engineering** drive these advancements forward.
Physics and Engineering
Physics and engineering are intricately linked disciplines that have revolutionized our understanding of the world and transformed it through practical applications. At the heart of this synergy lies the concept of frequency, a fundamental measure in both fields. Frequency, measured in Hertz (Hz), quantifies the number of oscillations or cycles per second in a wave or periodic phenomenon. This metric is crucial across various engineering and physics applications, enabling precise design, analysis, and optimization of systems. In physics, frequency is a cornerstone in understanding wave behavior, whether it involves mechanical waves like sound or electromagnetic waves such as light and radio waves. The study of frequency helps physicists delve into the properties of matter and energy, from the vibrational modes of molecules to the quantum mechanical behavior of particles. For instance, in quantum mechanics, the frequency of a photon is directly related to its energy, a relationship described by Planck's equation. This understanding has led to breakthroughs in fields like spectroscopy, where the analysis of light frequencies helps identify chemical compositions. In engineering, frequency plays a pivotal role in designing and optimizing systems. Electrical engineers rely heavily on frequency analysis to develop efficient power transmission systems, ensuring that electrical grids operate at optimal frequencies to minimize energy loss. In telecommunications, frequency allocation is critical for avoiding signal interference and ensuring clear communication channels. Mechanical engineers use frequency analysis to study the vibrational behavior of structures, predicting potential failures and improving the durability of machines and buildings. Aerospace engineers, too, utilize frequency analysis to understand the dynamic behavior of aircraft and spacecraft, ensuring stability and performance under various conditions. The practical uses of frequency extend beyond these traditional domains. In biomedical engineering, frequency analysis is used in medical imaging techniques such as MRI (Magnetic Resonance Imaging) and ultrasound, allowing for detailed diagnostics without invasive procedures. In materials science, the study of vibrational frequencies helps researchers understand material properties at the atomic level, leading to the development of new materials with tailored characteristics. Moreover, advancements in technology have made it possible to manipulate and control frequencies with unprecedented precision. For example, laser technology relies on the precise control of light frequencies to achieve coherent light sources, which are essential for applications ranging from cutting-edge medical treatments to high-speed data transmission. Similarly, in the field of nanotechnology, researchers are exploring ways to manipulate vibrational frequencies at the nanoscale to create novel devices with unique properties. In conclusion, the measurement of frequency is a unifying thread that weaves through both physics and engineering, enabling a deep understanding of natural phenomena and facilitating the development of innovative technologies. By leveraging this fundamental concept, scientists and engineers continue to push the boundaries of what is possible, transforming theoretical knowledge into practical solutions that enhance our daily lives. Whether it's optimizing energy transmission, developing advanced medical diagnostics, or creating new materials, the precise measurement and application of frequency remain indispensable tools in the pursuit of scientific and technological progress.
Music and Acoustics
Music and acoustics are intricately intertwined, forming the backbone of various disciplines that extend beyond the realm of entertainment. Acoustics, the study of sound and its properties, plays a crucial role in understanding how music is perceived, produced, and manipulated. In the context of music, acoustics delves into the physical properties of sound waves, including frequency, amplitude, and wavelength. Frequency, measured in Hertz (Hz), is particularly significant as it determines the pitch of a sound. For instance, a higher frequency corresponds to a higher pitch, while lower frequencies produce deeper tones. The practical applications of music and acoustics span multiple fields. In architecture, acousticians design concert halls and recording studios to optimize sound quality. They use principles such as reverberation time and sound absorption to create spaces where music can be appreciated in its purest form. In engineering, understanding acoustics is vital for developing audio equipment like microphones, speakers, and headphones. These devices rely on precise manipulation of sound waves to deliver high-quality audio. In medicine, acoustics is used in diagnostic tools such as ultrasound technology. Here, high-frequency sound waves are employed to create images of internal organs, aiding in the diagnosis of various health conditions. Additionally, music therapy leverages the emotional and psychological impact of music to treat mental health disorders and improve cognitive function. In environmental science, acoustics helps in monitoring wildlife populations through bioacoustics. By analyzing the sounds produced by animals, researchers can track population sizes, migration patterns, and even detect early signs of ecosystem distress. This field also extends to noise pollution studies, where understanding the impact of human-generated noise on wildlife habitats is crucial for conservation efforts. Furthermore, in materials science and physics, the study of acoustics contributes to the development of new materials with specific sound-absorbing or sound-reflecting properties. These materials are used in various applications ranging from soundproofing buildings to enhancing the performance of musical instruments. The intersection of music and acoustics also influences technology development. For example, audio signal processing algorithms rely heavily on acoustic principles to enhance or manipulate sound in real-time. These algorithms are used in everything from voice assistants to music streaming services, ensuring that the audio delivered is clear and distortion-free. In conclusion, the relationship between music and acoustics is multifaceted and far-reaching. By understanding how sound behaves and interacts with different environments and materials, researchers and practitioners across various disciplines can develop innovative solutions that enhance our lives in numerous ways. Whether it's designing better concert halls, creating more accurate medical imaging tools, or conserving wildlife habitats, the principles of acoustics play a pivotal role in making these advancements possible.
Telecommunications and Signal Processing
Telecommunications and signal processing are intricately linked disciplines that underpin the modern communication landscape, enabling the efficient transmission and reception of information across various mediums. Telecommunications, the backbone of global connectivity, relies heavily on signal processing to ensure that data is transmitted accurately and reliably. Signal processing involves the manipulation and analysis of signals to extract meaningful information, enhance signal quality, and optimize transmission efficiency. In telecommunications, this translates into several practical applications. For instance, in wireless communication systems such as cellular networks and satellite communications, signal processing techniques like modulation, demodulation, and error correction are crucial. Modulation allows information to be encoded onto carrier waves for transmission over long distances without significant loss of data integrity. Demodulation reverses this process at the receiving end, extracting the original information from the carrier wave. Error correction algorithms, such as those based on Reed-Solomon codes or turbo codes, detect and correct errors that may occur during transmission due to noise or interference. In addition to wireless communications, signal processing plays a vital role in wired networks like fiber optic systems. Here, techniques such as pulse shaping and equalization help mitigate distortions that occur during signal propagation through cables. This ensures that data packets are delivered with minimal latency and high fidelity. Beyond telecommunications, signal processing has far-reaching implications across multiple disciplines. In medicine, for example, signal processing is used in medical imaging technologies like MRI (Magnetic Resonance Imaging) and CT (Computed Tomography) scans. These technologies rely on sophisticated algorithms to reconstruct detailed images of the body from raw data captured by sensors. Similarly, in audio engineering, signal processing techniques such as filtering and compression are essential for enhancing sound quality in music production and live performances. In finance, signal processing is applied in algorithmic trading to analyze market trends and predict future price movements. By processing large datasets of historical market data, these algorithms can identify patterns that may not be apparent through manual analysis alone. Moreover, in environmental monitoring and climate science, signal processing is used to analyze data from sensors deployed to measure parameters such as temperature, humidity, and atmospheric pressure. This helps scientists understand complex environmental phenomena and make accurate predictions about weather patterns and climate changes. In summary, the synergy between telecommunications and signal processing drives innovation across diverse fields by enabling efficient data transmission and analysis. Whether it's ensuring clear voice calls over mobile networks or analyzing medical images for diagnostic purposes, these technologies form the foundation upon which many modern applications are built. Their practical uses span from enhancing communication systems to advancing scientific research, making them indispensable tools in today's interconnected world.