What Is The Charge Of A Proton
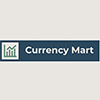
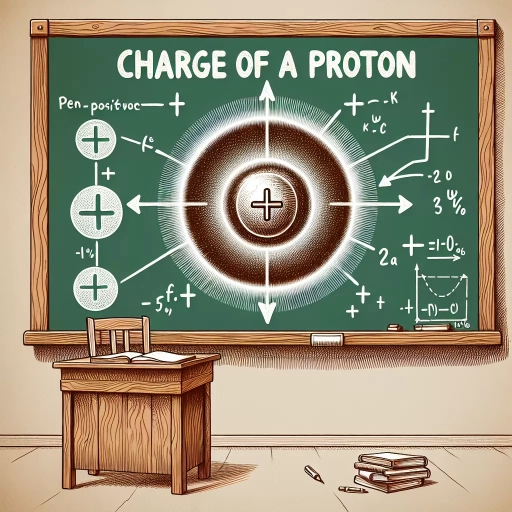
The charge of a proton is a fundamental concept in physics and chemistry, underpinning our understanding of atomic structure and the behavior of matter at its most basic level. This article delves into the intricacies of proton charge, exploring its significance across various scientific disciplines. We begin by examining the proton's role in atomic structure, highlighting how its positive charge interacts with electrons to form the nucleus of an atom. Next, we delve into the fundamental properties of the proton's charge, discussing its magnitude and how it compares to other subatomic particles. Finally, we explore the broader implications of proton charge in physics and chemistry, revealing how this property influences chemical reactions, nuclear stability, and the overall structure of molecules. By understanding these aspects, we gain a deeper appreciation for the proton's central role in the atomic framework. Let us start by Understanding the Proton's Role in Atomic Structure.
Understanding the Proton's Role in Atomic Structure
Understanding the proton's role in atomic structure is crucial for grasping the fundamental principles of chemistry and physics. At the heart of every atom lies the nucleus, where protons play a pivotal role. This article delves into three key aspects of protons: their relationship with the atomic number, their position and function within the nucleus, and their interaction with neutrons. Firstly, protons are directly linked to the atomic number of an element, which defines the element's identity in the periodic table. The number of protons in an atom's nucleus determines its chemical properties and how it interacts with other elements. Secondly, protons reside in the nucleus along with neutrons, forming the core of the atom. This positioning is essential for understanding atomic stability and the overall structure of matter. Lastly, the relationship between protons and neutrons is vital for comprehending nuclear stability and the diversity of isotopes within elements. By exploring these facets, we gain a deeper insight into how protons shape the very essence of atomic structure. Let us begin by examining how protons and atomic number are intricately connected, setting the stage for a comprehensive understanding of the proton's multifaceted role.
Protons and Atomic Number
Protons play a pivotal role in the atomic structure, and their presence is what defines an element's identity through its atomic number. The atomic number, a fundamental concept in chemistry and physics, is the number of protons present in the nucleus of an atom. This number determines the element's position in the periodic table and distinguishes it from other elements. For instance, hydrogen has an atomic number of 1 because it has one proton in its nucleus, while helium has an atomic number of 2 due to its two protons. The charge of a proton is a positive elementary charge, denoted as +e or +1.6 × 10^-19 coulombs. This positive charge is balanced by the negative charge of electrons orbiting the nucleus, ensuring that a neutral atom has an equal number of protons and electrons. The stability and chemical properties of an element are significantly influenced by this balance. For example, when an atom gains or loses electrons, it becomes an ion with a net positive or negative charge, altering its chemical behavior. In addition to defining an element's identity, protons also contribute to the overall mass of an atom. Although much lighter than neutrons and protons combined, electrons contribute negligibly to the atomic mass compared to the heavier protons and neutrons. The mass number of an atom, which is the sum of protons and neutrons in the nucleus, provides further insight into an element's isotopes—atoms of the same element with different numbers of neutrons. Understanding the role of protons in atomic structure is crucial for various scientific disciplines. In nuclear physics, the interaction between protons and neutrons within the nucleus is a key area of study, helping scientists understand nuclear stability and radioactive decay. In chemistry, knowing the number of protons allows chemists to predict chemical reactivity and bonding patterns, which are essential for synthesizing new compounds and understanding chemical reactions. Moreover, advancements in technology have made it possible to manipulate protons at a subatomic level. Techniques such as proton therapy in cancer treatment involve accelerating protons to high speeds to target and destroy cancer cells while minimizing damage to surrounding healthy tissue. This precision is only possible because of our deep understanding of proton behavior and their role in atomic structure. In conclusion, protons are not just positively charged particles within an atom's nucleus; they are the defining feature that distinguishes one element from another through their contribution to the atomic number. Their positive charge, mass contribution, and role in chemical properties make them a cornerstone of atomic structure. As science continues to evolve, our understanding of protons will remain fundamental to advancing our knowledge in physics, chemistry, and related fields.
Protons in the Nucleus
Protons, along with neutrons, form the nucleus of an atom, playing a crucial role in defining the atomic structure and properties of elements. Located at the center of the atom, protons are positively charged particles that contribute significantly to the overall charge and mass of the nucleus. Each proton carries a positive charge of +1 elementary charge, which is equal to 1.60217662 × 10^-19 coulombs. This positive charge is fundamental in determining the chemical identity of an element, as the number of protons in an atom's nucleus (atomic number) uniquely identifies each element on the periodic table. The presence of protons in the nucleus also influences the atomic mass, although their contribution is often overshadowed by the slightly heavier neutrons. Protons have a mass of approximately 1.67262171 × 10^-27 kilograms, which is roughly 1836 times that of an electron. The combined mass of protons and neutrons in the nucleus constitutes the atomic mass of an element, with protons contributing to about half of this total for most elements. One of the key roles of protons is their involvement in nuclear stability. The strong nuclear force, mediated by gluons, binds protons and neutrons together within the nucleus, overcoming the electrostatic repulsion between positively charged protons. This balance between attractive and repulsive forces ensures that the nucleus remains stable under normal conditions. However, when this balance is disrupted—either by an excess or deficiency of protons relative to neutrons—the nucleus can become unstable, leading to radioactive decay. In addition to their structural role, protons are involved in various nuclear reactions that are essential for understanding atomic phenomena. For instance, proton-proton chain reactions are central to the process of nuclear fusion in stars, including our Sun. These reactions involve the fusion of protons to form helium nuclei, releasing vast amounts of energy in the process. Understanding the role of protons in atomic structure is also crucial for applications in fields such as chemistry and physics. In chemistry, knowing the number of protons (atomic number) helps predict an element's chemical properties and reactivity. In physics, studying proton interactions provides insights into fundamental forces and the behavior of matter at subatomic levels. In summary, protons are indispensable components of atomic nuclei, contributing to both the charge and mass of atoms while ensuring nuclear stability through their interactions with neutrons. Their positive charge defines an element's identity on the periodic table, and their involvement in various nuclear processes underscores their importance in understanding atomic structure and phenomena.
Protons and Neutrons Relationship
In the intricate dance of atomic structure, protons and neutrons form a symbiotic relationship that underpins the very fabric of matter. Located within the nucleus of an atom, these subatomic particles play complementary roles that are crucial for the stability and identity of elements. Protons, positively charged particles with a mass of approximately 1 atomic mass unit (amu), are the primary determinants of an element's chemical properties. The number of protons in an atom's nucleus defines its atomic number, which uniquely identifies each element on the periodic table. For instance, hydrogen has one proton, helium has two, and so on. Neutrons, on the other hand, are neutral particles with a mass slightly greater than that of protons. They do not carry any electric charge but contribute significantly to the overall mass of the nucleus. The interplay between protons and neutrons is vital for maintaining nuclear stability. In most atoms, the number of neutrons is roughly equal to or slightly greater than the number of protons. This balance is essential because it allows the strong nuclear force—a fundamental force of nature that holds protons and neutrons together—to overcome the electrostatic repulsion between positively charged protons. The relationship between protons and neutrons also gives rise to isotopes—atoms of the same element that have different numbers of neutrons. For example, carbon-12 and carbon-14 are isotopes of carbon; they both have six protons but differ in their neutron count (six neutrons in carbon-12 and eight neutrons in carbon-14). This variation in neutron number affects the atomic mass but not the chemical properties, as these are determined solely by the number of protons. Furthermore, the proton-neutron relationship is critical in nuclear reactions such as fusion and fission. In fusion reactions, light nuclei combine to form heavier nuclei, releasing energy in the process. This is exemplified by the sun's core where hydrogen nuclei (protons) fuse to form helium nuclei. Conversely, fission involves splitting heavy nuclei into lighter ones, also releasing energy but often accompanied by radioactive decay. Understanding this intricate relationship between protons and neutrons is fundamental to grasping atomic structure and its implications across various fields such as chemistry, physics, and engineering. It underscores how these subatomic particles work together to create stable atoms that form the basis of all matter around us. By recognizing how protons define an element's identity while neutrons contribute to its mass and stability, we gain a deeper appreciation for the intricate mechanisms governing our universe at its most fundamental level. This knowledge not only enhances our comprehension of natural phenomena but also drives technological advancements in fields like nuclear energy and materials science. In summary, the relationship between protons and neutrons is a cornerstone of atomic structure, enabling us to understand how elements are formed, how they interact with each other, and how they can be manipulated to achieve specific outcomes. This symbiotic bond between positively charged protons and neutral neutrons is a testament to the elegant complexity of nature at its smallest scales.
The Charge of a Proton: Fundamental Properties
The charge of a proton is a fundamental property that underpins our understanding of atomic structure and the behavior of matter at its most basic level. This intrinsic characteristic is pivotal in various scientific disciplines, including physics and chemistry. To delve into the essence of proton charge, it is crucial to explore three key aspects: the definition of proton charge, the perspective offered by quantum mechanics, and the experimental verification that solidifies our knowledge. Firstly, understanding the **Definition of Proton Charge** is essential as it sets the foundation for comprehending how protons interact within atoms and molecules. This definition will clarify what exactly is meant by the term "charge" in the context of protons. Secondly, examining the **Quantum Mechanics Perspective** provides insights into how protons behave at the subatomic level, revealing the intricate dynamics governed by quantum principles. This perspective helps in understanding the probabilistic nature of proton interactions. Lastly, **Experimental Verification** is critical as it validates theoretical models through empirical evidence. Experiments have consistently shown that the charge of a proton is a constant, fundamental quantity that remains unchanged across different environments. By exploring these three facets, we can gain a comprehensive understanding of the charge of a proton. Let us begin by defining what we mean by proton charge, as this foundational concept is the cornerstone of our discussion.
Definition of Proton Charge
The charge of a proton is a fundamental property that underpins the structure and behavior of atoms, molecules, and ultimately, all matter. At its core, the proton charge is a quantifiable measure of the positive electric charge carried by protons, which are among the three primary constituents of atomic nuclei, alongside neutrons and electrons. This charge is a cornerstone of quantum mechanics and classical electromagnetism, defining how protons interact with other charged particles. To be precise, the charge of a proton is approximately \(1.602 \times 10^{-19}\) coulombs. This value is a constant of nature and is denoted by the symbol \(e\), often referred to as the elementary charge. The proton's positive charge is exactly equal in magnitude but opposite in sign to the negative charge of an electron. This balance is crucial for the stability of atoms; in a neutral atom, the number of protons (which determines the atomic number) is equal to the number of electrons, ensuring that the overall charge of the atom is zero. The proton's charge plays a pivotal role in various physical phenomena. For instance, it influences the chemical properties of elements by determining how atoms interact with each other through electrostatic forces. In chemical bonding, protons in the nucleus attract electrons from other atoms, leading to the formation of covalent bonds or ionic bonds. Additionally, the proton's charge is essential in nuclear reactions such as fusion and fission, where changes in the number of protons within an atom's nucleus result in different elements. From a theoretical perspective, understanding the proton's charge has been a milestone in the development of modern physics. The discovery of this fundamental constant has been refined over centuries through experiments and theoretical work by scientists such as Robert Millikan and J.J. Thomson. Today, precise measurements of the proton's charge continue to be important for advancing our understanding of particle physics and for calibrating scientific instruments used in research. In practical applications, knowledge of the proton's charge is vital in fields like chemistry, materials science, and engineering. For example, in semiconductor technology, controlling the number of protons (and thus the charge) in materials allows for the creation of devices with specific electrical properties. Similarly, in medical treatments like proton therapy for cancer, precise control over proton beams relies on a deep understanding of their charge and how it interacts with tissue. In summary, the charge of a proton is not just a numerical value but a fundamental aspect of physics that governs atomic interactions and underpins many natural phenomena. Its precise measurement and understanding have been instrumental in advancing scientific knowledge and technological innovations across various disciplines. As research continues to refine our understanding of subatomic particles and their properties, the proton's charge remains an indispensable concept in both theoretical and applied sciences.
Quantum Mechanics Perspective
In the realm of quantum mechanics, the charge of a proton is not just a fundamental property but a cornerstone that underpins our understanding of the atomic structure and the behavior of matter at the smallest scales. From a quantum perspective, the proton's charge is a quantized entity, meaning it is an intrinsic and unchanging attribute that cannot be divided into smaller units. This quantization is a hallmark of quantum mechanics, where physical properties such as energy, spin, and charge are discrete rather than continuous. Quantum mechanics introduces the concept of wave-particle duality, suggesting that particles like protons exhibit both wave-like and particle-like behavior. This duality is crucial for understanding how protons interact within atomic nuclei and how they contribute to the overall charge of an atom. The charge of a proton, denoted as \(+e\) (where \(e\) is the elementary charge), is a fundamental constant in physics that plays a pivotal role in determining the chemical properties of elements. It is this precise charge that allows protons to attract electrons, forming neutral atoms, and it is the imbalance of this charge that leads to the formation of ions. The principles of quantum mechanics also shed light on the stability of protons within atomic nuclei. According to the Heisenberg Uncertainty Principle, it is impossible to know both the position and momentum of a proton with infinite precision simultaneously. This principle, along with the Pauli Exclusion Principle, helps explain why protons and neutrons occupy specific energy levels within the nucleus, contributing to its stability. The strong nuclear force, mediated by gluons in the context of quantum chromodynamics (QCD), is responsible for holding protons and neutrons together despite their positive charges. Furthermore, quantum field theory (QFT) provides a more nuanced understanding of the proton's charge by describing it as an emergent property arising from the interactions of quarks and gluons. Protons are composed of three quarks—two up quarks and one down quark—each carrying a fraction of the elementary charge. The combination of these quark charges results in the net positive charge of the proton. This QFT perspective highlights the dynamic nature of the proton's charge, which is not just a static property but an outcome of complex interactions at the subatomic level. In summary, the charge of a proton from a quantum mechanics perspective is a fundamental, quantized property that underpins our understanding of atomic structure and nuclear stability. It is through the lens of quantum mechanics that we can appreciate the intricate dance of particles and forces that give rise to this essential attribute, making it a cornerstone in our comprehension of the physical world.
Experimental Verification
Experimental verification is a cornerstone in the scientific process, particularly when determining fundamental properties such as the charge of a proton. This rigorous approach ensures that theoretical models and hypotheses are grounded in empirical evidence. To verify the charge of a proton, scientists employ various experimental techniques that leverage the principles of electromagnetism and particle physics. One of the most significant experiments in this context is the Millikan oil drop experiment, conducted by Robert Millikan in the early 20th century. In this experiment, Millikan used an electric field to suspend tiny oil droplets in mid-air, allowing him to measure the charge on individual droplets with remarkable precision. By carefully controlling the electric field and observing the motion of the droplets, Millikan was able to calculate the elementary charge, which is the charge carried by a single proton. This experiment not only provided a precise value for the proton's charge but also demonstrated the quantized nature of electric charge. Another crucial method involves the use of mass spectrometry. This technique separates ions based on their mass-to-charge ratio, enabling scientists to determine the charge of a proton by comparing it with known masses and charges of other particles. For instance, by analyzing the deflection of protons in a magnetic field, researchers can calculate the proton's charge relative to its mass, further validating the results obtained from other experiments. In addition to these classical methods, modern particle physics experiments at high-energy colliders, such as the Large Hadron Collider (LHC), provide further verification. Here, protons are accelerated to nearly the speed of light and then made to collide, producing a plethora of subatomic particles. By analyzing the properties and interactions of these particles, physicists can infer the charge of the proton with even greater precision. These experiments also allow for the study of proton-proton interactions at the quantum level, reinforcing our understanding of the proton's charge within the framework of quantum field theory. The synergy between theoretical predictions and experimental verification is essential for establishing the fundamental properties of particles like the proton. Theoretical models, such as quantum electrodynamics (QED), predict the behavior of charged particles under various conditions. Experimental verification of these predictions not only confirms our understanding but also drives the refinement of theoretical models. For example, precise measurements of the proton's charge have implications for our understanding of atomic structure and the behavior of matter at the atomic and subatomic level. In conclusion, experimental verification plays a pivotal role in determining the charge of a proton. Through a combination of historical experiments like Millikan's oil drop experiment, modern mass spectrometry techniques, and cutting-edge particle physics research, scientists have been able to establish the proton's charge with high accuracy. These experiments underscore the importance of empirical evidence in validating theoretical models and highlight the ongoing quest for precision in understanding the fundamental properties of matter. By continually refining our measurements and testing our theories against experimental data, we deepen our understanding of the universe at its most basic level.
Implications of Proton Charge in Physics and Chemistry
The proton charge is a fundamental concept in both physics and chemistry, with far-reaching implications that shape our understanding of various phenomena. At its core, the positive charge of a proton influences electromagnetic interactions, which are crucial for understanding how particles interact at the atomic and subatomic levels. This foundational aspect also plays a pivotal role in chemical bonding and reactions, as it dictates the formation of molecules and the reactivity of elements. Furthermore, the proton charge is essential for nuclear stability and decay, influencing the balance within atomic nuclei and determining the stability or instability of isotopes. By examining these three key areas—electromagnetic interactions, chemical bonding and reactions, and nuclear stability and decay—we can gain a comprehensive insight into the profound impact of proton charge on the behavior of matter. Let us begin by delving into the realm of electromagnetic interactions, where the proton's positive charge sets the stage for a multitude of physical phenomena.
Electromagnetic Interactions
Electromagnetic interactions are a fundamental aspect of understanding the charge of a proton and its implications in physics and chemistry. These interactions arise from the electromagnetic force, one of the four fundamental forces of nature, which governs how charged particles interact with each other. The proton, being positively charged, plays a crucial role in these interactions. When two charged particles are brought close to each other, they experience an electromagnetic force described by Coulomb's Law. This law states that the force between two charges is proportional to the product of their magnitudes and inversely proportional to the square of the distance between them. In the context of atomic structure, electromagnetic interactions are essential for holding electrons in orbit around the nucleus, where protons reside. The positive charge of protons attracts negatively charged electrons, forming stable atoms through electrostatic attraction. In chemistry, these interactions are pivotal for understanding chemical bonding. Ionic bonds form when electrons are transferred between atoms, resulting in ions with opposite charges that attract each other electromagnetically. Covalent bonds, on the other hand, involve sharing of electron pairs between atoms, which is stabilized by the electrostatic attraction between the positively charged nuclei and the negatively charged electrons. The precise charge of a proton (approximately +1.602 × 10^-19 Coulombs) is critical in determining the strength and nature of these chemical bonds. Moreover, electromagnetic interactions involving protons are central to various physical phenomena. For instance, in nuclear physics, the electromagnetic force helps explain certain aspects of nuclear stability and decay processes. Although the strong nuclear force is primarily responsible for holding protons and neutrons together within the nucleus, electromagnetic interactions contribute to the overall stability by influencing the arrangement of nucleons. In addition to their role in atomic and molecular structures, electromagnetic interactions involving protons have significant technological implications. In particle accelerators, protons are accelerated to high speeds using electromagnetic fields, allowing scientists to study subatomic particles and forces at unprecedented energies. In medical applications such as proton therapy for cancer treatment, precise control over proton beams relies on understanding and manipulating electromagnetic interactions to target tumors accurately. The study of electromagnetic interactions also extends into cosmology and astrophysics. The charge of a proton affects the behavior of plasmas in stars and other celestial bodies, influencing processes like nuclear fusion reactions that power stellar life cycles. Understanding these interactions is crucial for modeling cosmic phenomena and interpreting observational data from space missions. In conclusion, the charge of a proton is not just a fundamental constant but a cornerstone for understanding a wide range of electromagnetic interactions that underpin both chemical bonding and various physical phenomena. These interactions are essential for explaining atomic stability, chemical reactivity, and numerous technological applications, making the precise determination of proton charge a cornerstone of modern physics and chemistry.
Chemical Bonding and Reactions
Chemical bonding and reactions are fundamental processes in both physics and chemistry, deeply influenced by the charge of a proton. The proton, with its positive charge, plays a crucial role in the formation of atoms and molecules. In atomic structure, protons reside in the nucleus along with neutrons, while electrons orbit around it. The number of protons in an atom determines its chemical identity, making each element unique. Chemical bonding occurs when atoms share or exchange electrons to achieve a stable electronic configuration. This stability is often achieved by filling the outermost energy level of an atom, which typically requires eight electrons (the octet rule). The positive charge of protons attracts negatively charged electrons, holding them in their orbits. In covalent bonds, atoms share pairs of electrons to form molecules like water (H₂O) and methane (CH₄). Ionic bonds form when one or more electrons are transferred from one atom to another, resulting in ions with opposite charges that attract each other, as seen in sodium chloride (NaCl). The charge of a proton also influences chemical reactions. In acid-base reactions, for instance, hydrogen ions (H⁺) play a key role. These ions are essentially protons that have been stripped of their electrons. When an acid donates a proton, it forms a conjugate base, while the base that accepts the proton becomes a conjugate acid. This proton transfer is central to many biochemical processes, including those in living organisms. Furthermore, the proton's charge affects the reactivity of molecules. Electronegativity, which is the tendency of an atom to attract electrons towards itself in a covalent bond, is influenced by the number of protons in an atom's nucleus. Atoms with higher numbers of protons (and thus higher atomic numbers) tend to be more electronegative because their stronger nuclear charge pulls electrons closer to the nucleus. In addition to these fundamental aspects, the proton's charge has significant implications for advanced chemical processes such as catalysis and polymerization. Catalysts often work by stabilizing transition states or intermediates through electrostatic interactions involving protons and other charged species. Polymerization reactions, which involve the formation of large molecules from smaller monomers, can be initiated or accelerated by proton transfer mechanisms. Understanding the role of proton charge in chemical bonding and reactions is essential for various fields beyond basic chemistry. It underpins pharmaceutical research where drug design relies on understanding how molecules interact at a molecular level. It also drives technological advancements in materials science and energy storage, where new materials with specific properties are developed based on their atomic and molecular structures. In conclusion, the positive charge of a proton is pivotal in shaping the principles of chemical bonding and reactions. From the simplest covalent bonds to complex biochemical processes, the proton's influence permeates every level of chemical interaction. This fundamental aspect of atomic structure not only explains why elements behave as they do but also underpins many technological and scientific advancements across diverse fields.
Nuclear Stability and Decay
Nuclear stability and decay are fundamental concepts that underscore the intricate balance and dynamics within atomic nuclei, directly influenced by the charge of protons. The stability of a nucleus is largely determined by the interplay between the strong nuclear force, which binds protons and neutrons together, and the electrostatic repulsion between positively charged protons. This delicate balance is crucial because it dictates whether a nucleus will remain stable or undergo radioactive decay. Protons, with their positive charge, play a pivotal role in this equilibrium. The number of protons in a nucleus defines the element's identity and influences its stability. Nuclei with too many or too few neutrons relative to protons are generally unstable and tend to decay into more stable configurations. For instance, nuclei with an excess of neutrons may undergo beta decay, where a neutron is converted into a proton, an electron, and an antineutrino, thereby reducing the neutron-to-proton ratio and achieving greater stability. The charge of a proton also affects nuclear stability through its impact on the strong nuclear force. This force, mediated by gluons, is responsible for holding quarks together inside protons and neutrons and binding these nucleons within the nucleus. However, the strong force has a limited range and cannot counteract the electrostatic repulsion between protons indefinitely. As the number of protons increases, so does the electrostatic repulsion, making it more challenging for the strong force to maintain nuclear cohesion. This is why heavier elements, with more protons, are generally less stable and more prone to radioactive decay. Understanding nuclear stability and decay has significant implications in both physics and chemistry. In physics, it underpins our comprehension of nuclear reactions, including fission and fusion processes that are critical for energy production and astrophysical phenomena. For example, nuclear fission, where a heavy nucleus splits into lighter nuclei, releases a substantial amount of energy due to the reduction in electrostatic repulsion among protons. Conversely, nuclear fusion, such as that occurring in stars, involves the combination of light nuclei into heavier ones, overcoming the electrostatic barrier through immense temperatures and pressures. In chemistry, the stability of nuclei influences the availability and properties of elements. Radioactive decay processes can lead to the formation of new elements or isotopes, which may have unique chemical properties. For instance, certain isotopes produced through radioactive decay are used in medical applications, such as in cancer treatment and imaging. Additionally, understanding nuclear stability helps chemists predict the reactivity and behavior of elements, particularly those with unstable nuclei that may undergo spontaneous decay. In conclusion, the charge of a proton is a critical factor in determining nuclear stability and decay. The balance between the strong nuclear force and electrostatic repulsion among protons dictates whether a nucleus remains stable or undergoes radioactive transformations. This fundamental principle has far-reaching implications across physics and chemistry, influencing our understanding of nuclear reactions, energy production, astrophysical phenomena, and the properties and applications of elements.