What Is Complete Dominance
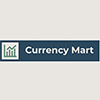
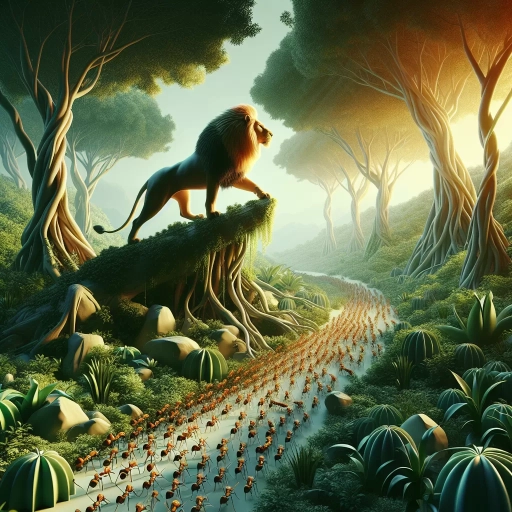
Complete dominance is a fundamental concept in genetics that describes the relationship between different alleles of a gene, where one allele completely masks the effect of the other. This phenomenon is crucial for understanding how traits are inherited and expressed in organisms. In this article, we will delve into the definition and concept of complete dominance, exploring its underlying genetic mechanisms and how it differs from other types of allelic interactions. We will also examine real-world examples and case studies that illustrate complete dominance in action, highlighting its significance in various biological contexts. Finally, we will discuss the implications and applications of complete dominance, including its role in breeding programs, genetic counseling, and the broader field of genetics. By understanding complete dominance, we gain insights into the intricate world of genetic inheritance and its practical applications. Let us begin by defining and conceptualizing complete dominance to lay the groundwork for our exploration.
Definition and Concept of Complete Dominance
Complete dominance is a fundamental concept in genetics that describes the relationship between two alleles of a gene, where one allele completely masks the effect of the other. This phenomenon is crucial for understanding the inheritance of traits in organisms. The article delves into the definition and concept of complete dominance, exploring its genetic basis, phenotypic expression, and how it differs from incomplete dominance. By examining the genetic basis of complete dominance, we uncover the molecular mechanisms that underpin this phenomenon, including the interaction between dominant and recessive alleles. The phenotypic expression section elucidates how these genetic interactions manifest in observable traits. Additionally, the article highlights the key differences between complete and incomplete dominance, providing clarity on when each type of dominance occurs. Understanding these aspects is essential for grasping the complexities of genetic inheritance. Transitioning to the genetic basis of complete dominance, we will explore how specific alleles interact to produce dominant and recessive traits, setting the stage for a deeper dive into the molecular underpinnings of this genetic principle.
Genetic Basis of Complete Dominance
The genetic basis of complete dominance is rooted in the principles of Mendelian genetics, where one allele of a gene completely masks the effect of the other allele. This phenomenon occurs when the dominant allele (often denoted by an uppercase letter, e.g., "B") produces a functional product that is sufficient to produce the dominant phenotype, regardless of whether the individual is homozygous (BB) or heterozygous (Bb) for the gene. Conversely, the recessive allele (denoted by a lowercase letter, e.g., "b") either does not produce a functional product or produces one that is insufficient to manifest the recessive phenotype in the presence of the dominant allele. At the molecular level, complete dominance can arise from several mechanisms. One common scenario is where the dominant allele encodes a fully functional protein, while the recessive allele encodes a non-functional or partially functional protein. For example, in the case of flower color in peas, the dominant "B" allele codes for an enzyme necessary for pigment production, resulting in purple flowers. The recessive "b" allele, on the other hand, codes for a non-functional version of this enzyme, leading to white flowers. When an individual is heterozygous (Bb), the presence of one functional "B" allele is enough to produce the enzyme and thus purple flowers, illustrating complete dominance. Another mechanism involves gene regulation, where the dominant allele may influence the expression of other genes in a way that masks the effect of the recessive allele. For instance, if the dominant allele upregulates a pathway that leads to a specific trait, the presence of this allele will ensure that the pathway is active regardless of the presence of the recessive allele. In addition to these molecular mechanisms, complete dominance can also be influenced by dosage effects. In some cases, having one copy of the dominant allele provides sufficient gene product to achieve the dominant phenotype, while having two copies does not significantly enhance the effect. This is seen in traits like tallness in pea plants, where one "T" allele is enough to produce the tall phenotype. Understanding the genetic basis of complete dominance is crucial for predicting inheritance patterns and for applications in genetics and breeding programs. It allows scientists and breeders to predict how traits will be expressed in offspring based on the genotypes of the parents, which is essential for selecting desirable traits in crops and livestock. In summary, the genetic basis of complete dominance involves the interaction between alleles at a single gene locus, where the dominant allele produces a sufficient amount of functional product to mask the effect of the recessive allele. This can be due to differences in protein function, gene regulation, or dosage effects, and it forms the foundation for understanding many inherited traits in organisms.
Phenotypic Expression in Complete Dominance
In the context of complete dominance, phenotypic expression refers to the observable traits or characteristics of an organism that result from the interaction of its genotype and the environment. When one allele (a variant of a gene) completely masks the effect of another allele, this is known as complete dominance. For instance, in a simple Mendelian inheritance pattern involving two alleles (let's denote them as "B" for the dominant allele and "b" for the recessive allele), the presence of just one copy of the dominant allele (B) will result in the dominant phenotype being expressed, regardless of whether the genotype is BB or Bb. This means that both homozygous dominant (BB) and heterozygous (Bb) individuals will display the same dominant trait, while only homozygous recessive (bb) individuals will express the recessive trait. To illustrate this concept further, consider a classic example involving flower color in pea plants. If "B" represents the allele for purple flowers (dominant) and "b" represents the allele for white flowers (recessive), then plants with genotypes BB or Bb will have purple flowers, while only those with genotype bb will have white flowers. This clear-cut distinction in phenotypic expression highlights how complete dominance operates: the dominant allele dictates the observable trait, overshadowing any influence from the recessive allele. Understanding phenotypic expression in complete dominance is crucial for predicting genetic outcomes in breeding programs and for diagnosing genetic disorders. It helps geneticists and breeders anticipate how traits will be inherited and expressed across generations. Moreover, this concept underpins many practical applications in agriculture, where desirable traits such as disease resistance or improved yield can be selectively bred into crops by leveraging complete dominance. In summary, phenotypic expression in complete dominance is characterized by the dominant allele's ability to fully express its trait, masking any effect of the recessive allele. This results in a consistent and predictable pattern of trait inheritance, making it a fundamental principle in genetics with significant practical implications.
Difference from Incomplete Dominance
In the context of genetics, the concept of complete dominance is often contrasted with incomplete dominance to highlight their distinct mechanisms and outcomes. Complete dominance occurs when one allele of a gene completely masks the effect of the other allele, resulting in only one phenotype being expressed. For instance, in the classic example of flower color in snapdragons, the red flower color (R) is completely dominant over the white flower color (r), meaning that plants with the genotype RR or Rr will have red flowers, while only rr plants will have white flowers. In contrast, incomplete dominance does not involve one allele completely masking the other. Instead, both alleles contribute to the phenotype, leading to a blended or intermediate effect. Using the same flower color example, if we consider a scenario where neither red nor white is completely dominant, we might observe a pink flower color in plants with the Rr genotype. Here, the presence of both alleles results in a phenotype that is intermediate between the two parental types. The key difference lies in how the alleles interact. In complete dominance, one allele's effect is so strong that it overrides the other, while in incomplete dominance, both alleles have an equal or nearly equal influence on the final phenotype. This distinction is crucial for understanding genetic inheritance patterns and predicting offspring phenotypes. For example, in breeding programs, knowing whether a trait exhibits complete or incomplete dominance can significantly impact the selection and breeding strategies employed. Furthermore, incomplete dominance can lead to more variability within a population because it allows for the expression of multiple phenotypes based on different genotypes. This variability can be advantageous in certain environments, as it provides more options for natural selection to act upon. On the other hand, complete dominance tends to reduce variability by masking one allele's effect entirely, which can be beneficial in scenarios where a specific trait is highly advantageous. Understanding these differences is essential for genetic studies and applications in fields such as agriculture, medicine, and conservation biology. By recognizing whether a trait follows complete or incomplete dominance, researchers and breeders can make informed decisions about how to manipulate genetic traits to achieve desired outcomes. Thus, while complete dominance simplifies the prediction of phenotypes due to its straightforward masking effect, incomplete dominance introduces complexity but also greater genetic diversity and potential for adaptation.
Examples and Case Studies of Complete Dominance
The concept of complete dominance is a fundamental principle in genetics, where one allele of a gene completely masks the effect of the other allele. This phenomenon is observed across various biological systems, providing valuable insights into inheritance patterns. In this article, we will delve into three key areas that illustrate complete dominance: human traits, plant and animal examples, and historical studies. We will begin by examining **Human Traits Exhibiting Complete Dominance**, where we explore how certain genetic traits in humans follow the rules of complete dominance, influencing physical characteristics such as eye color and blood type. Next, we will look at **Plant and Animal Examples**, highlighting instances in other organisms where complete dominance is evident, such as flower color in plants and coat color in animals. Finally, **Historical Studies on Complete Dominance** will discuss landmark research that has contributed to our understanding of this genetic principle. By exploring these diverse examples, we gain a comprehensive understanding of how complete dominance shapes the genetic landscape of living organisms. Transitioning to our first focus area, let's start with **Human Traits Exhibiting Complete Dominance** to see how this genetic principle manifests in our own species.
Human Traits Exhibiting Complete Dominance
Human traits exhibiting complete dominance are genetic characteristics where one allele of a gene completely masks the effect of the other allele, resulting in a dominant phenotype. This concept is fundamental in genetics and can be observed in various human traits. For instance, the ability to roll one's tongue is a classic example of complete dominance. Here, the dominant allele (R) for tongue rolling masks the recessive allele (r), meaning individuals with either RR or Rr genotypes can roll their tongues, while those with rr genotypes cannot. Another example is the presence of freckles, where the dominant allele (F) for freckles will always result in the presence of freckles in individuals with FF or Ff genotypes, while those with ff genotypes will not have freckles. In the case of blood type, the ABO blood group system also demonstrates complete dominance. The A and B alleles are codominant to each other but dominant over the O allele. Individuals with AA or AO genotypes have type A blood, those with BB or BO genotypes have type B blood, and those with AB genotypes have type AB blood. However, individuals with OO genotypes have type O blood, illustrating how the absence of A or B alleles results in a recessive phenotype. A significant case study involves the genetic disorder Huntington's disease, which is caused by an expansion of CAG repeats in the Huntingtin gene. This disorder exhibits complete dominance because a single copy of the mutated allele is sufficient to cause the disease. Individuals who inherit one mutated allele (either from their mother or father) will develop Huntington's disease, regardless of whether they also inherit a normal allele. Furthermore, certain physical traits like earlobe attachment also follow this pattern. Free earlobes are dominant (E), while attached earlobes are recessive (e). Individuals with EE or Ee genotypes have free earlobes, while those with ee genotypes have attached earlobes. These examples and case studies highlight how complete dominance influences the expression of various human traits, from simple physical characteristics to complex genetic disorders. Understanding these principles is crucial for predicting inheritance patterns and diagnosing genetic conditions. By examining these traits and their genetic basis, scientists can better comprehend human genetics and its implications for health and disease.
Plant and Animal Examples
In the context of complete dominance, several plant and animal examples illustrate this genetic principle clearly. One of the most well-known examples is the pea plant experiments conducted by Gregor Mendel. Mendel observed that when he crossed a tall pea plant (dominant trait) with a short pea plant (recessive trait), all the offspring were tall. This demonstrated that the tall trait completely dominated the short trait, resulting in no intermediate height in the first generation. Another example is the flower color in snapdragons; when a red-flowered snapdragon (dominant) is crossed with a white-flowered snapdragon (recessive), all the offspring have red flowers, showing complete dominance of the red color over the white. In animals, complete dominance can be seen in the coat color of mice. When a black mouse (dominant) is bred with a white mouse (recessive), all the offspring will have black coats because the gene for black coat color completely dominates the gene for white coat color. Similarly, in cattle, the gene for hornedness is dominant over the gene for hornlessness. When a horned cow is bred with a hornless cow, all the offspring will be horned, illustrating complete dominance. These examples highlight how complete dominance operates at the genetic level to produce specific phenotypes without any blending of traits. For instance, in humans, the gene for freckles is dominant over the gene for no freckles. If one parent has freckles and the other does not, their children are likely to have freckles because the dominant gene for freckles will be expressed. Understanding complete dominance is crucial for predicting genetic outcomes in breeding programs and for explaining why certain traits appear consistently in offspring. It also underscores the importance of Mendelian genetics in modern biology and genetics research. By studying these examples, scientists can better comprehend how genes interact to produce observable characteristics and how these interactions influence the diversity of life on Earth. Moreover, complete dominance has practical applications in agriculture and animal husbandry. For example, farmers can selectively breed crops or livestock to ensure desirable traits are consistently passed down to future generations. This selective breeding relies on the principle of complete dominance to guarantee that dominant traits such as disease resistance or high yield are expressed in offspring. In conclusion, the examples from pea plants, snapdragons, mice, cattle, and humans provide robust illustrations of complete dominance in action. These case studies not only validate Mendel's laws but also demonstrate the widespread applicability of genetic principles across different species and contexts. By examining these examples closely, we gain a deeper understanding of how genes control traits and how this knowledge can be harnessed for various practical purposes.
Historical Studies on Complete Dominance
Historical studies on complete dominance have been pivotal in understanding the genetic principles that govern inheritance patterns. The concept of complete dominance was first introduced by Gregor Mendel in the 19th century through his experiments with pea plants. Mendel observed that when he crossed purebred plants with different traits, such as tall and short stature or green and yellow seeds, the offspring exhibited only one of the parental traits in the first generation. This led him to propose the law of segregation, where each pair of alleles (different forms of a gene) separates during gamete formation, resulting in offspring that are either homozygous (having two copies of the same allele) or heterozygous (having one copy of each allele). In cases of complete dominance, one allele (the dominant allele) masks the effect of the other allele (the recessive allele), leading to a single observable trait in heterozygous individuals. One of the most illustrative examples from Mendel's work is the study on flower color. When he crossed purebred pea plants with red flowers (RR) and purebred pea plants with white flowers (rr), all the offspring in the first generation had red flowers (Rr), demonstrating that the red allele was dominant over the white allele. This principle has been consistently supported by subsequent genetic studies across various organisms, including humans. For instance, the genetics of blood type in humans follows a similar pattern, where the A and B alleles are codominant but the O allele is recessive to both A and B. Historical case studies also highlight how complete dominance plays a crucial role in agricultural genetics. In breeding programs, understanding which traits are dominant or recessive allows scientists to predict and control the inheritance of desirable characteristics. For example, in corn breeding, the trait for kernel color is influenced by multiple genes but often exhibits complete dominance. Breeders can use this knowledge to develop new varieties with specific kernel colors that are more resistant to pests or have improved nutritional content. Moreover, historical studies on complete dominance have implications beyond agriculture. In medical genetics, understanding the dominance relationships between alleles can help in diagnosing and managing genetic disorders. For instance, sickle cell anemia is caused by a recessive allele; individuals who are heterozygous for this allele (carriers) do not exhibit the disease but can pass it to their offspring if both parents are carriers. This knowledge has been crucial for genetic counseling and public health initiatives aimed at reducing the incidence of such diseases. In conclusion, historical studies on complete dominance have laid the foundation for modern genetics by elucidating how genes interact to produce observable traits. These findings have been consistently validated across different species and have significant practical applications in fields such as agriculture, medicine, and biotechnology. The continued exploration of these principles ensures that our understanding of genetic inheritance remains robust and informative.
Implications and Applications of Complete Dominance
The concept of complete dominance in genetics has far-reaching implications and diverse applications across various fields. In breeding programs, complete dominance plays a crucial role in selecting desirable traits and predicting offspring phenotypes, thereby enhancing the efficiency and precision of genetic selection. Additionally, its impact on genetic counseling is significant, as it helps in assessing the risk of inherited diseases and providing accurate genetic advice to families. Furthermore, complete dominance has substantial applications in biotechnology, where it is used to develop genetically modified organisms with specific characteristics. Understanding complete dominance is essential for leveraging these benefits, and this article will delve into each of these areas to provide a comprehensive overview. Starting with its role in breeding programs, we will explore how complete dominance influences the selection and propagation of desirable traits, setting the stage for a deeper examination of its broader genetic and biotechnological implications.
Role in Breeding Programs
In the context of breeding programs, complete dominance plays a crucial role in shaping the genetic makeup of offspring. Complete dominance occurs when one allele of a gene completely masks the effect of the other allele, resulting in a dominant phenotype. This principle is fundamental in selective breeding, where breeders aim to enhance desirable traits and eliminate undesirable ones. For instance, in plant breeding, complete dominance can be used to introduce disease resistance or improve yield. By selecting for the dominant allele that confers these beneficial traits, breeders can ensure that the desired characteristics are consistently expressed in subsequent generations. Similarly, in animal husbandry, complete dominance helps breeders to select for traits such as coat color, size, or fertility, thereby improving the overall quality and performance of the stock. The predictability of complete dominance allows breeders to make informed decisions about which individuals to breed, ensuring that the offspring will exhibit the desired traits. This precision is particularly valuable in programs aimed at conserving endangered species or developing new crop varieties, where every genetic decision can have significant implications for the long-term viability and success of the breeding program. Additionally, understanding complete dominance helps breeders avoid unintended consequences, such as the loss of genetic diversity, by ensuring that recessive alleles are not inadvertently eliminated from the gene pool. Overall, the application of complete dominance in breeding programs enhances the efficiency and effectiveness of genetic selection, leading to improved outcomes in agriculture, conservation, and biotechnology.
Impact on Genetic Counseling
The impact of genetic counseling is profound and multifaceted, particularly in the context of complete dominance. Genetic counseling plays a crucial role in helping individuals and families understand and manage genetic conditions that exhibit complete dominance. Complete dominance occurs when one allele of a gene completely masks the effect of the other allele, resulting in a dominant phenotype. For instance, in conditions like Huntington's disease, where the dominant allele leads to the development of the disease, genetic counseling is essential. Genetic counselors provide detailed information about the risks and probabilities associated with passing on a dominant allele to offspring. They help individuals understand their genetic status and the likelihood that their children may inherit the condition. This knowledge is critical for family planning and decision-making. For example, if one parent has Huntington's disease, there is a 50% chance that each child will inherit the dominant allele and develop the disease. Genetic counselors can guide families through these complex probabilities and help them make informed decisions about reproductive options. Moreover, genetic counseling extends beyond risk assessment to include emotional support and psychological guidance. Families dealing with genetic conditions often face significant emotional challenges, including anxiety, fear, and grief. Genetic counselors are trained to provide empathetic support and connect families with appropriate resources and support groups. This holistic approach ensures that individuals receive comprehensive care that addresses both the medical and psychological aspects of their situation. In addition to individual and family counseling, genetic counseling has broader implications for public health. By identifying and understanding genetic conditions that exhibit complete dominance, healthcare providers can develop targeted screening programs and early intervention strategies. For example, genetic screening for conditions like BRCA1 and BRCA2 (which are associated with an increased risk of breast and ovarian cancer) can lead to early detection and preventive measures, significantly improving health outcomes. Furthermore, advances in genetic counseling have led to the development of preimplantation genetic diagnosis (PGD) and prenatal testing. These technologies allow for the identification of genetic conditions in embryos or fetuses, enabling parents to make informed decisions about whether to proceed with a pregnancy. This application of genetic counseling not only helps families avoid the birth of children with severe genetic conditions but also reduces the societal burden associated with long-term care for these conditions. In conclusion, the impact of genetic counseling on individuals, families, and society as a whole is substantial. By providing accurate information, emotional support, and access to advanced diagnostic technologies, genetic counseling empowers individuals to make informed decisions about their health and reproductive choices. As our understanding of genetics continues to evolve, the role of genetic counseling will become even more critical in navigating the complexities of complete dominance and other genetic phenomena.
Applications in Biotechnology
Biotechnology, a field that integrates biology and technology, has far-reaching implications and diverse applications that significantly impact various sectors of society. One of the foundational concepts in genetics, complete dominance, plays a crucial role in these applications. Complete dominance occurs when one allele of a gene completely masks the effect of the other allele, leading to a dominant phenotype. This principle is pivotal in several biotechnological applications. In **agriculture**, complete dominance is utilized to develop crops with desirable traits such as pest resistance, drought tolerance, and enhanced nutritional content. For instance, genetic engineers can introduce dominant alleles that confer resistance to specific pests or diseases, thereby reducing the need for pesticides and improving crop yields. This not only enhances food security but also reduces environmental impact. In **medicine**, understanding complete dominance helps in the diagnosis and treatment of genetic disorders. Many genetic diseases are caused by dominant mutations where a single copy of the mutated gene is enough to cause the disease. Knowing this allows for precise genetic testing and counseling, enabling families to make informed decisions about their health. Additionally, biotechnology companies use this knowledge to develop targeted therapies that can mitigate or cure these conditions. **Pharmaceuticals** also benefit from the concept of complete dominance. Biotechnologists use this principle to engineer microorganisms that produce therapeutic proteins and drugs. For example, bacteria can be genetically modified to produce insulin, a life-saving drug for diabetics, by ensuring that the dominant allele responsible for insulin production is expressed. In **forensic science**, complete dominance aids in DNA profiling and paternity testing. By analyzing genetic markers where one allele dominates over another, forensic scientists can identify individuals and determine familial relationships with high accuracy. Moreover, **bioremediation**—the use of living organisms to clean up pollutants—relies on genetic modifications that often involve dominant alleles. Microorganisms can be engineered to degrade toxic substances more efficiently by introducing dominant genes that enhance their metabolic capabilities. Lastly, **synthetic biology** leverages complete dominance to design new biological pathways and circuits. By carefully selecting and combining dominant alleles, researchers can create novel biological systems that perform specific functions, such as producing biofuels or detecting environmental toxins. In summary, the principle of complete dominance is a cornerstone in various biotechnological applications, from agriculture and medicine to pharmaceuticals, forensic science, bioremediation, and synthetic biology. Understanding how dominant alleles interact and influence phenotypes allows scientists to develop innovative solutions that improve human health, environmental sustainability, and overall quality of life.