What Is Cell Specialization
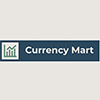
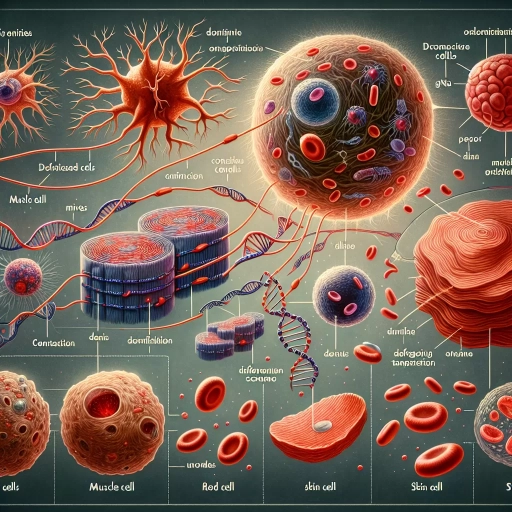
Cell specialization is a fundamental concept in biology that underpins the complexity and diversity of life. It refers to the process by which cells differentiate into specific types, each with unique structures and functions tailored to perform particular roles within an organism. This specialization is crucial for the development, growth, and maintenance of multicellular organisms, allowing them to adapt to various environments and ensure overall survival. In this article, we will delve into the intricacies of cell specialization, beginning with an **Introduction to Cell Specialization**, where we explore the basic principles and importance of this biological phenomenon. We will then examine the **Mechanisms of Cell Specialization**, discussing how genetic and environmental factors drive cell differentiation. Finally, we will highlight **Examples and Applications of Cell Specialization**, illustrating how this process manifests in different organisms and its practical implications in fields such as medicine and biotechnology. By understanding these aspects, we gain a deeper appreciation for the intricate mechanisms that govern life at the cellular level. Let us start by exploring the foundational concepts in our **Introduction to Cell Specialization**.
Introduction to Cell Specialization
Cell specialization is a fundamental concept in biology that underpins the complexity and diversity of life. It is the process by which cells become adapted to perform specific functions, enabling organisms to develop and maintain their intricate structures and functions. Understanding cell specialization is crucial because it highlights the importance of cellular diversity in maintaining health and function. This article delves into the definition and importance of cell specialization, exploring how specialized cells contribute to the overall well-being of an organism. We will also examine the historical context and discovery of cell specialization, tracing the key milestones that have shaped our current understanding. Additionally, we will discuss the basic principles of cellular differentiation, explaining how cells transition from a general state to a specialized one. By grasping these concepts, readers will gain a comprehensive insight into the fascinating world of cell specialization, setting the stage for a deeper exploration of this critical biological phenomenon in the following sections on **Introduction to Cell Specialization**.
Definition and Importance of Cell Specialization
Cell specialization, a fundamental concept in biology, refers to the process by which cells within an organism differentiate to perform specific functions. This specialization is crucial for the complexity and efficiency of multicellular organisms. At its core, cell specialization involves the expression of unique sets of genes that enable cells to develop distinct structures and functions tailored to their roles within the organism. For instance, nerve cells (neurons) are specialized for rapid transmission of electrical signals, while muscle cells (myocytes) are optimized for contraction and movement. This diversity in cellular function allows for the intricate coordination necessary for maintaining life processes such as digestion, respiration, and reproduction. The importance of cell specialization cannot be overstated. It underpins the hierarchical organization of tissues, organs, and organ systems that collectively form a functional organism. Without specialization, cells would lack the efficiency and precision required to carry out complex biological tasks. For example, in the human body, red blood cells are specialized to transport oxygen efficiently due to their high concentration of hemoglobin and lack of a nucleus, which maximizes their oxygen-carrying capacity. Similarly, pancreatic beta cells are specialized to produce insulin, regulating blood sugar levels with precision. This division of labor ensures that each cell type contributes optimally to the overall health and survival of the organism. Moreover, cell specialization is a key factor in the adaptability and resilience of organisms. Specialized cells can respond more effectively to environmental changes or internal needs. For instance, immune cells like T-cells and B-cells are specialized to recognize and combat pathogens, providing a robust defense mechanism against infections. The loss or dysfunction of specialized cells can lead to severe health issues; for example, diabetes results from the failure of pancreatic beta cells to produce sufficient insulin. In addition to its functional significance, cell specialization also highlights the remarkable plasticity and developmental potential of cells. During embryonic development, stem cells undergo differentiation into various specialized cell types through a series of tightly regulated genetic and epigenetic changes. Understanding these processes has profound implications for regenerative medicine and tissue engineering, where scientists aim to harness the power of cell specialization to repair damaged tissues or replace dysfunctional cells. In conclusion, cell specialization is a cornerstone of biological complexity, enabling multicellular organisms to achieve a level of functional sophistication that is not possible in single-celled organisms. Its importance extends beyond mere efficiency; it is essential for the very survival and adaptability of life as we know it. As research continues to unravel the intricacies of cellular differentiation and function, our appreciation for the critical role of cell specialization in maintaining life's delicate balance only deepens.
Historical Context and Discovery
The historical context and discovery of cell specialization are deeply intertwined with the evolution of cellular biology. The journey began in the 17th century when Antonie van Leeuwenhoek, often referred to as the "Father of Microbiology," first observed cells using his handcrafted microscopes. However, it was not until the 19th century that the concept of cell specialization began to take shape. Theodor Schwann and Matthias Jakob Schleiden's groundbreaking work in the 1830s led to the formulation of the cell theory, which posits that all living organisms are composed of cells and that cells are the basic units of life. This foundational theory laid the groundwork for understanding how cells could differ in structure and function. As microscopy techniques improved, scientists like Rudolf Virchow and Walther Flemming made significant contributions. Virchow's dictum "Omnis cellula e cellula" (every cell comes from a cell) emphasized the importance of cellular division and differentiation. Flemming's discovery of mitosis in the late 19th century provided a mechanistic understanding of how cells divide, which was crucial for grasping how specialized cells arise. The early 20th century saw a surge in research on cellular differentiation, particularly with the work of embryologists like Hans Spemann and Hilde Mangold. Their experiments on amphibian embryos demonstrated the presence of organizer regions that could induce specific developmental pathways, leading to the concept of morphogenetic fields and the idea that cells are not isolated entities but interact within complex systems to achieve specialization. The advent of molecular biology in the mid-20th century revolutionized our understanding of cell specialization. The discovery of DNA structure by James Watson and Francis Crick in 1953 and subsequent advances in genetics and molecular biology tools allowed scientists to explore the genetic and molecular mechanisms underlying cellular differentiation. This era saw the identification of key regulatory genes and signaling pathways that orchestrate the process of cell specialization. Today, our understanding of cell specialization is multifaceted, involving insights from genetics, epigenetics, and systems biology. Modern techniques such as single-cell RNA sequencing have enabled researchers to map the transcriptomic landscapes of individual cells, revealing intricate patterns of gene expression that define specialized cell types. This wealth of knowledge has profound implications for fields such as regenerative medicine, cancer research, and developmental biology, highlighting the dynamic and highly regulated nature of cellular specialization in living organisms. The historical trajectory from early microscopic observations to current molecular and genomic analyses underscores the continuous pursuit of understanding how cells become specialized, a quest that remains at the heart of cellular biology.
Basic Principles of Cellular Differentiation
Cellular differentiation is a fundamental process in the development and maintenance of complex organisms, where cells acquire specialized functions and structures to perform specific roles. This intricate process is governed by a set of basic principles that ensure the precise and coordinated transformation of undifferentiated cells into their mature, functional forms. At its core, cellular differentiation involves the regulation of gene expression, where specific genes are activated or repressed to produce the unique set of proteins required for the cell's specialized function. This genetic regulation is often mediated by transcription factors, which bind to DNA sequences to either enhance or inhibit the transcription of target genes. The process begins with the commitment of a cell to a particular lineage, a decision influenced by both intrinsic cellular signals and extrinsic environmental cues. Once committed, the cell undergoes a series of molecular changes that include alterations in chromatin structure, epigenetic modifications, and changes in the expression of key regulatory genes. These changes collectively drive the cell towards its final differentiated state, characterized by the acquisition of specific morphological features and functional capabilities. Another critical principle is the concept of cellular plasticity, which allows cells to revert to earlier stages of differentiation or even switch between different lineages under certain conditions. This flexibility is essential for tissue repair and regeneration, as well as for the development of certain diseases such as cancer, where cells can lose their differentiated state and regain proliferative potential. The microenvironment also plays a crucial role in cellular differentiation, providing physical and biochemical signals that guide the cell's developmental path. Cell-cell interactions, growth factors, and the extracellular matrix all contribute to creating a niche that supports or inhibits specific differentiation pathways. Furthermore, cellular differentiation is tightly linked with cell cycle regulation. Differentiated cells often exit the cell cycle and enter a quiescent state, focusing their energy on performing their specialized functions rather than proliferating. This balance between proliferation and differentiation is essential for maintaining tissue homeostasis and preventing uncontrolled growth. In summary, the basic principles of cellular differentiation involve precise genetic regulation, commitment to specific lineages, cellular plasticity, influence of the microenvironment, and coordination with cell cycle control. These principles work in concert to ensure that cells acquire the necessary specialized traits to contribute effectively to the overall function of an organism. Understanding these principles is crucial for appreciating how cell specialization underpins the complexity and diversity of life.
Mechanisms of Cell Specialization
Cell specialization is a fundamental process in biology, enabling cells to acquire unique functions that contribute to the complexity and diversity of life. This intricate process involves a series of highly regulated mechanisms that guide cells from a general state to a specialized one. At the heart of cell specialization are three critical components: gene expression and regulation, signaling pathways, and epigenetic modifications. Gene expression and regulation ensure that specific genes are turned on or off to produce the necessary proteins for specialized functions. Signaling pathways play a crucial role in coordinating the differentiation process by transmitting external signals that influence cellular behavior. Additionally, epigenetic modifications fine-tune gene expression without altering the DNA sequence itself, providing a layer of control that is essential for maintaining cellular identity. Understanding these mechanisms is essential for appreciating how cells become specialized and how this specialization contributes to the overall health and function of organisms. In this article, we will delve into these key aspects to provide a comprehensive introduction to cell specialization.
Gene Expression and Regulation
Gene expression and regulation are pivotal mechanisms that underpin cell specialization, enabling cells to adopt diverse functions despite sharing the same genetic blueprint. At its core, gene expression involves the translation of genetic information encoded in DNA into proteins, which are the functional units of cells. This process is tightly regulated to ensure that each cell type expresses only the genes necessary for its specific role. For instance, in muscle cells, genes related to muscle contraction are highly expressed, while in nerve cells, genes involved in neurotransmission are prioritized. The regulation of gene expression occurs at multiple levels, starting from transcription—the process by which DNA is transcribed into RNA. Transcription factors, which are proteins that bind to specific DNA sequences near the genes they regulate, play a crucial role in initiating or inhibiting transcription. These factors can either enhance or repress gene expression based on the cell's needs and environmental cues. Post-transcriptional regulation further refines this process by controlling mRNA stability, splicing, and translation efficiency. MicroRNAs and siRNAs, for example, can bind to mRNA molecules and prevent their translation into proteins. Epigenetic modifications also significantly influence gene expression without altering the DNA sequence itself. Methylation of DNA and histone modification can either compact chromatin structure, making genes inaccessible to transcription machinery, or open it up, facilitating gene expression. These epigenetic changes are heritable through cell divisions but reversible, allowing for dynamic regulation of gene expression in response to developmental signals or environmental changes. In the context of cell specialization, differential gene expression ensures that each cell type acquires its unique set of proteins necessary for its specialized functions. For example, during embryonic development, stem cells undergo a series of differentiation steps where specific sets of genes are activated or repressed to guide them towards becoming particular cell types such as neurons, muscle cells, or epithelial cells. This precise regulation of gene expression is critical for the development and maintenance of tissue-specific functions and overall organismal health. Moreover, dysregulation of gene expression can lead to various diseases including cancer, where aberrant transcriptional programs drive uncontrolled cell proliferation and loss of cellular identity. Understanding the mechanisms of gene expression and regulation thus not only sheds light on how cells specialize but also provides insights into potential therapeutic strategies for treating diseases associated with disrupted cellular differentiation. In summary, gene expression and its regulation are fundamental to the process of cell specialization, allowing cells to express specific genes that define their functional identities. The intricate interplay between transcriptional control, post-transcriptional regulation, and epigenetic modifications ensures that each cell type performs its unique role within the organism, underscoring the complexity and precision of cellular differentiation.
Role of Signaling Pathways in Differentiation
**Role of Signaling Pathways in Differentiation** Signaling pathways play a pivotal role in the process of cell differentiation, which is a cornerstone of cell specialization. Differentiation is the process by which a cell becomes specialized in structure and function to perform a specific role. This intricate process is orchestrated by a complex interplay of signaling pathways that ensure precise and timely expression of genes necessary for the acquisition of specialized cellular functions. At the heart of differentiation are signaling cascades that involve the activation of receptors on the cell surface by specific ligands. These receptors can be tyrosine kinase receptors, G-protein coupled receptors, or other types, each triggering distinct downstream signaling events. For instance, the Notch signaling pathway is crucial in many developmental contexts, influencing cell fate decisions by regulating transcription factors that drive or inhibit differentiation. Similarly, the Wnt/β-catenin pathway is vital for embryonic development and tissue homeostasis, modulating gene expression to guide cells towards specific lineages. The activation of these pathways leads to a cascade of intracellular events involving kinases, phosphatases, and transcription factors. These molecules interact in a highly regulated manner to control the expression of target genes. For example, in the case of neuronal differentiation, the activation of the PI3K/Akt pathway promotes survival and growth, while the MAPK/ERK pathway regulates proliferation and differentiation. The precise coordination of these pathways ensures that cells acquire the necessary phenotypic characteristics to fulfill their specialized roles. Moreover, signaling pathways are not isolated entities but rather part of a larger network that integrates multiple inputs to achieve a coherent cellular response. Cross-talk between different pathways allows for fine-tuning and ensures that the differentiation process is adaptable to changing environmental conditions. This integration is exemplified by the interplay between the BMP (Bone Morphogenetic Protein) and FGF (Fibroblast Growth Factor) pathways during embryonic development, where their combined activity orchestrates the formation of complex tissues. In addition to their role in developmental biology, signaling pathways are also critical in adult tissues where they maintain tissue homeostasis and facilitate regeneration. For instance, in the hematopoietic system, signaling through the JAK/STAT pathway is essential for the differentiation of hematopoietic stem cells into various blood cell types. Dysregulation of these pathways can lead to developmental abnormalities or diseases such as cancer, highlighting their importance in maintaining cellular homeostasis. In summary, signaling pathways are the molecular conduits that translate extracellular cues into intracellular responses, driving cells towards specialized fates. Their precise regulation and integration are essential for the proper differentiation of cells, underscoring their central role in mechanisms of cell specialization. Understanding these pathways not only provides insights into developmental biology but also opens avenues for therapeutic interventions aimed at correcting differentiation defects in various diseases.
Epigenetic Modifications and Their Impact
Epigenetic modifications play a pivotal role in the mechanisms of cell specialization, enabling cells to adopt diverse functions without altering the underlying DNA sequence. These modifications involve chemical changes to DNA or histone proteins, which wrap DNA into chromatin, thereby influencing gene expression. One key type of epigenetic modification is DNA methylation, where methyl groups are added to specific DNA sequences, typically resulting in gene silencing. This process is crucial for cell differentiation, as it allows certain genes to be turned off while others are activated, leading to the specialized functions of different cell types. Another significant epigenetic mechanism is histone modification. Histones can be modified through various processes such as acetylation, methylation, phosphorylation, and ubiquitination. These modifications can either relax or compact chromatin structure, thereby regulating access to transcription factors and other regulatory proteins. For instance, histone acetylation generally leads to a more open chromatin structure, facilitating gene transcription and promoting the expression of genes necessary for specific cellular functions. Chromatin remodeling complexes also contribute to epigenetic regulation by reorganizing nucleosomes to expose or hide DNA sequences. This dynamic process ensures that genes required for cell specialization are accessible to the transcriptional machinery while irrelevant genes are sequestered. Additionally, non-coding RNAs, such as microRNAs and long non-coding RNAs, can modulate gene expression by binding to specific mRNAs or chromatin regions, further fine-tuning the epigenetic landscape. The impact of these epigenetic modifications on cell specialization is profound. They allow for the precise control of gene expression necessary for the development and maintenance of diverse cell types within an organism. For example, during embryonic development, epigenetic changes guide the differentiation of stem cells into various lineages such as neurons, muscle cells, and blood cells. In adult tissues, epigenetic modifications help maintain cellular identity and function, ensuring that cells perform their specialized roles efficiently. Moreover, dysregulation of epigenetic mechanisms has been implicated in various diseases, including cancer and neurological disorders. In cancer, aberrant DNA methylation and histone modifications can lead to the silencing of tumor suppressor genes or the activation of oncogenes. Understanding these epigenetic alterations is crucial for developing targeted therapies that can restore normal gene expression patterns. In summary, epigenetic modifications are essential for cell specialization by dynamically regulating gene expression without altering the DNA sequence. These mechanisms ensure that cells acquire and maintain their specialized functions, which are vital for the proper functioning of tissues and organs. The intricate interplay between different types of epigenetic modifications underscores their critical role in cellular differentiation and highlights the importance of continued research into these complex processes.
Examples and Applications of Cell Specialization
Cell specialization is a fundamental concept in biology, where cells adapt to perform specific functions, enhancing the efficiency and complexity of organisms. This phenomenon is crucial for the proper functioning of various body systems, as seen in the diverse roles of specialized cells within the human body. For instance, nerve cells transmit signals, muscle cells facilitate movement, and epithelial cells form protective barriers. The potential of cell specialization extends beyond these basic functions, particularly in the realm of medicine. Stem cells, with their ability to differentiate into multiple cell types, hold significant promise for treating a wide range of diseases. Their versatility could revolutionize medical treatments by providing new avenues for tissue repair and regeneration. Furthermore, understanding cell specialization has profound implications for disease treatment and research, enabling scientists to develop targeted therapies and better comprehend the underlying mechanisms of various disorders. In this article, we will delve into these aspects, exploring specialized cells in human body systems, the potential of stem cells in medicine, and the implications for disease treatment and research. This journey begins with an **Introduction to Cell Specialization**, where we will lay the groundwork for understanding how cells become specialized and why this process is so vital for life.
Specialized Cells in Human Body Systems
In the intricate tapestry of the human body, cell specialization is a cornerstone that enables the efficient functioning of various systems. Specialized cells, each with unique structures and functions, work in harmony to maintain homeostasis and ensure the overall health of the organism. For instance, in the nervous system, neurons are specialized to transmit and process information through electrical and chemical signals. These cells have elongated axons that facilitate rapid signal transmission, while dendrites receive signals from other neurons, allowing for complex neural networks to form. In contrast, the muscular system relies on muscle cells (fibers) that are designed for contraction and relaxation. Skeletal muscle fibers are multinucleated and contain myofibrils, which are essential for voluntary movements, whereas smooth muscle cells lack striations and are involved in involuntary actions such as peristalsis in the digestive tract. The circulatory system is another prime example where specialized cells play a crucial role. Red blood cells (erythrocytes) are disk-shaped and lack a nucleus, maximizing their surface area for oxygen transport. White blood cells (leukocytes), including neutrophils, lymphocytes, and monocytes, are specialized for immune responses; they recognize and eliminate pathogens through mechanisms like phagocytosis and antibody production. In the digestive system, epithelial cells lining the gut are specialized for absorption; they have microvilli that increase the surface area for nutrient uptake. Pancreatic islet cells produce hormones such as insulin and glucagon to regulate blood sugar levels, illustrating how cell specialization contributes to metabolic balance. The respiratory system also benefits from specialized cells. Alveolar type I cells in the lungs are thin and flat, facilitating gas exchange between the air we breathe and the bloodstream. Alveolar type II cells produce surfactant, a substance that reduces surface tension in the alveoli, making it easier to expand the lungs during inhalation. In the integumentary system, keratinocytes in the skin produce keratin, providing structural support and protection against external factors like UV radiation and pathogens. Furthermore, cell specialization is evident in the reproductive system. Sperm cells are highly specialized with a streamlined head containing genetic material and a long flagellum for motility, ensuring successful fertilization. Egg cells (oocytes) are large and contain nutrients necessary for early embryonic development. These examples underscore how cell specialization allows for the diverse range of functions necessary for human survival and well-being. By optimizing their structures and functions, specialized cells ensure that each system operates efficiently, contributing to the remarkable complexity and resilience of the human body. This intricate specialization is a testament to the evolutionary adaptations that have enabled humans to thrive in a wide range of environments.
Stem Cells and Their Potential in Medicine
Stem cells, with their unique ability to differentiate into various cell types, hold immense potential in the field of medicine. These cells, which can be derived from embryos, adult tissues, or induced from other cell types, offer a versatile tool for treating a wide range of diseases and injuries. One of the most promising applications of stem cells is in regenerative medicine. For instance, in the treatment of heart disease, stem cells can be used to repair damaged heart tissue by differentiating into functional cardiac cells, thereby improving heart function and reducing the risk of further complications. Similarly, in neurodegenerative diseases such as Parkinson's and Alzheimer's, stem cells can be engineered to replace damaged neurons, potentially halting disease progression and restoring cognitive function. In orthopedics, stem cells are being explored for their ability to regenerate bone and cartilage tissue, offering new hope for patients suffering from osteoarthritis or severe bone fractures. The use of mesenchymal stem cells (MSCs) in particular has shown significant promise due to their anti-inflammatory properties and capacity to differentiate into bone, cartilage, and other connective tissues. Additionally, hematopoietic stem cells are crucial in bone marrow transplantation for treating blood-related disorders like leukemia and lymphoma by replenishing the patient's blood-forming system. Stem cells also play a critical role in tissue engineering, where they are combined with biomaterials to create functional tissue substitutes. For example, researchers are working on developing bioengineered skin substitutes using stem cells to treat severe burns and chronic wounds. Furthermore, the potential of stem cells in gene therapy is being explored, where genetically modified stem cells can be used to correct genetic defects at the cellular level, offering a potential cure for inherited diseases. Moreover, induced pluripotent stem cells (iPSCs), which are generated from adult cells reprogrammed back into an embryonic-like state, offer a personalized approach to medicine. These cells can be used to model diseases in vitro, allowing for better understanding of disease mechanisms and testing of new drugs. This personalized medicine approach also reduces the risk of immune rejection associated with traditional transplantation methods. In summary, the versatility and regenerative capabilities of stem cells make them a cornerstone in modern medical research. Their applications span across various medical disciplines, from cardiovascular health to neurology and orthopedics, offering new avenues for treatment and potential cures for previously intractable diseases. As research continues to advance, the full potential of stem cells in medicine is likely to be realized, transforming the way we approach healthcare and disease management.
Implications for Disease Treatment and Research
The implications of cell specialization for disease treatment and research are profound and multifaceted. Understanding how cells specialize allows scientists to develop targeted therapies that exploit the unique characteristics of specific cell types. For instance, in cancer treatment, recognizing the specialized functions of cancer cells versus normal cells enables the design of drugs that selectively kill cancerous cells while sparing healthy ones. This precision reduces side effects and improves treatment efficacy. Similarly, in regenerative medicine, knowing how stem cells differentiate into various specialized cell types helps researchers develop strategies to repair or replace damaged tissues, such as in the treatment of heart disease or spinal cord injuries. In neurological disorders like Parkinson's disease, understanding the specialization of dopamine-producing neurons informs the development of therapies aimed at restoring or replacing these cells. Gene therapy, which involves introducing genes into cells to correct genetic defects, relies heavily on cell specialization principles to ensure that the therapeutic genes are expressed in the appropriate cell types. Furthermore, the study of cell specialization has led to significant advancements in immunotherapy, where immune cells are engineered to recognize and target specific cancer cells, thereby enhancing the body's natural defense mechanisms. From a research perspective, cell specialization provides a rich framework for understanding disease mechanisms at the cellular level. By studying how cells specialize under normal conditions, scientists can identify aberrant specialization patterns that contribute to disease states. This knowledge can be used to develop diagnostic markers and predictive models for various diseases. Additionally, the ability to induce specific cell types from stem cells in vitro has revolutionized drug testing and toxicity screening, allowing for more accurate and ethical preclinical trials. In summary, the understanding and application of cell specialization have transformed the landscape of disease treatment and research. By leveraging the unique properties of specialized cells, scientists can develop more effective and targeted therapies, improve diagnostic capabilities, and advance our understanding of disease mechanisms. This field continues to evolve rapidly, promising even more innovative solutions for combating a wide range of diseases in the future.