What Is Alternative Splicing
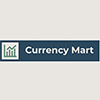
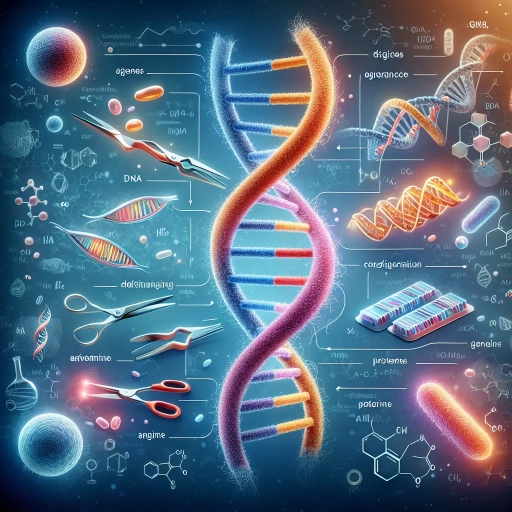
Alternative splicing is a fundamental process in molecular biology that significantly expands the diversity of proteins produced by a single gene, thereby enhancing the complexity and versatility of cellular functions. This intricate mechanism allows for the generation of multiple protein isoforms from a single pre-mRNA transcript, each potentially having distinct roles and functions. Understanding alternative splicing is crucial for grasping its mechanisms and regulation, which involve a delicate interplay of splicing factors, RNA sequences, and other cellular components. The biological and clinical implications of alternative splicing are profound, influencing various physiological processes and contributing to the pathogenesis of numerous diseases. In this article, we will delve into the introduction to alternative splicing, exploring its basic principles and how it operates. We will then examine the mechanisms and regulation that govern this process, highlighting the key players and pathways involved. Finally, we will discuss the biological and clinical implications of alternative splicing, shedding light on its significance in both normal cellular function and disease states. By understanding these aspects, we can appreciate the full scope of alternative splicing's impact on life at the molecular level. Let us begin with an introduction to alternative splicing.
Introduction to Alternative Splicing
Alternative splicing is a fundamental process in molecular biology that significantly enhances the complexity and diversity of gene expression. This intricate mechanism allows a single gene to produce multiple proteins, each with potentially distinct functions, by selectively including or excluding different exons during the splicing of pre-mRNA. To fully appreciate the significance of alternative splicing, it is essential to delve into its definition and basic concepts, which form the foundation of understanding this phenomenon. Historically, the discovery of alternative splicing marked a pivotal moment in the field of genetics, challenging the traditional one-gene-one-protein hypothesis and opening new avenues for research into gene regulation. This historical context provides valuable insights into how our understanding of gene expression has evolved over time. The importance of alternative splicing in gene expression cannot be overstated. It plays a crucial role in developmental processes, tissue-specific gene regulation, and the generation of protein isoforms that can have different cellular functions. By exploring these aspects, we can better comprehend how alternative splicing contributes to the rich tapestry of cellular diversity. To begin our exploration, let us first define and understand the basic concepts underlying alternative splicing, which will serve as a cornerstone for grasping its broader implications and significance.
Definition and Basic Concepts
Alternative splicing, a fundamental process in molecular biology, is the mechanism by which a single gene can produce multiple distinct proteins. This versatility is achieved through the differential inclusion or exclusion of exons (coding regions) during the splicing of pre-mRNA transcripts. At its core, alternative splicing involves the selective removal of introns (non-coding regions) and the joining of exons in various combinations, leading to diverse mRNA isoforms that can be translated into different proteins. To understand this concept fully, it is essential to grasp the basic steps involved in RNA splicing. Initially, the transcription of a gene results in a pre-mRNA molecule that contains both exons and introns. The splicing process, mediated by the spliceosome—a complex of RNA and proteins—excises introns and joins exons together. Alternative splicing occurs when different combinations of exons are included or excluded from the final mRNA transcript, often regulated by specific splicing factors that bind to splice sites or other regulatory elements within the pre-mRNA. The definition of alternative splicing encompasses several key aspects: **1) Exon skipping**, where an exon is entirely omitted from the mature mRNA; **2) Intron retention**, where an intron is not removed and becomes part of the mature mRNA; **3) Alternative 5' or 3' splice sites**, where different splice sites within an exon or intron are used; and **4) Mutually exclusive exons**, where two or more exons are never included in the same mRNA molecule. These variations allow a single gene to generate a wide array of proteins with potentially different functions, subcellular localizations, or stabilities. The significance of alternative splicing lies in its ability to expand the proteomic diversity of an organism without increasing the number of genes. This process is particularly prevalent in higher eukaryotes, such as humans, where it is estimated that over 90% of genes undergo alternative splicing. The resulting protein isoforms can have distinct biological activities, contributing to cellular complexity and adaptability. For instance, alternative splicing can influence protein-protein interactions, enzymatic activity, and subcellular localization, thereby playing critical roles in development, tissue specificity, and disease pathogenesis. In summary, alternative splicing is a sophisticated regulatory mechanism that enhances the functional repertoire of genes by generating multiple protein products from a single genetic template. Understanding the definition and basic concepts of alternative splicing is crucial for appreciating its role in shaping the complexity of eukaryotic genomes and its implications for human health and disease. This foundational knowledge sets the stage for exploring the intricate mechanisms and biological outcomes associated with this vital process.
Historical Context and Discovery
The discovery of alternative splicing is deeply rooted in the historical context of molecular biology, particularly during the latter half of the 20th century. This period saw a significant surge in understanding the intricacies of gene expression and the mechanisms by which cells generate diverse proteins from a limited number of genes. The 1970s marked a pivotal era with the advent of recombinant DNA technology, which allowed scientists to manipulate and analyze DNA sequences with unprecedented precision. This technological breakthrough enabled researchers to delve into the structure and function of genes, leading to the identification of introns—non-coding regions within genes that are removed during the RNA splicing process. One of the earliest and most influential discoveries related to alternative splicing was made by Richard Roberts and Phillip Sharp, who independently identified split genes in 1977. Their work revealed that genes are not continuous sequences but are instead interrupted by introns, which are excised and spliced out during the maturation of messenger RNA (mRNA). This groundbreaking finding laid the foundation for understanding how a single gene can produce multiple distinct mRNAs through different splicing patterns, a phenomenon that would later be termed alternative splicing. The subsequent decades witnessed rapid advancements in sequencing technologies and the development of sophisticated computational tools, which facilitated the comprehensive analysis of genomic data. These advancements allowed researchers to catalog and study the prevalence of alternative splicing across various organisms, revealing its widespread occurrence and functional significance. For instance, studies on model organisms such as Drosophila melanogaster (fruit flies) and Caenorhabditis elegans (nematode worms) provided insights into how alternative splicing contributes to developmental processes, tissue specificity, and cellular responses to environmental cues. The Human Genome Project, completed in 2003, further underscored the importance of alternative splicing by highlighting that the human genome contains fewer than 20,000 protein-coding genes—far fewer than anticipated. This discrepancy between gene number and protein diversity was largely resolved by recognizing that alternative splicing allows a single gene to encode multiple proteins, thereby increasing the functional complexity of the genome. Today, it is estimated that over 90% of human genes undergo alternative splicing, making it a critical regulatory mechanism in eukaryotic gene expression. Understanding the historical context of alternative splicing's discovery not only appreciates the scientific milestones but also underscores the ongoing evolution of our knowledge. From the initial identification of split genes to the current high-throughput sequencing and bioinformatics approaches, each step has built upon previous discoveries, enriching our comprehension of this complex biological process. As research continues to unravel the intricacies of alternative splicing, it is clear that this phenomenon remains a vibrant area of study, offering insights into gene regulation, cellular differentiation, and potential therapeutic targets for diseases. The journey from the early days of molecular biology to the present has been marked by significant advancements, and it is this historical context that provides a rich backdrop for exploring the multifaceted world of alternative splicing.
Importance in Gene Expression
Gene expression is the fundamental process by which the information encoded in a gene's DNA is converted into a functional product, such as a protein or RNA molecule. This intricate process is crucial for the development, growth, and maintenance of all living organisms. The importance of gene expression lies in its ability to regulate cellular functions, allowing cells to respond to environmental changes, differentiate into specialized types, and maintain homeostasis. At the heart of gene expression is the transcription of DNA into RNA, followed by translation of RNA into proteins. However, the complexity of gene expression extends beyond these basic steps due to mechanisms like alternative splicing. Alternative splicing, a key aspect of post-transcriptional regulation, significantly enhances the diversity of gene expression. This process involves the selective inclusion or exclusion of exons (coding regions) during the splicing of pre-mRNA transcripts, resulting in multiple mature mRNA molecules from a single gene. This versatility allows a single gene to produce a variety of proteins with different functions, thereby increasing the functional complexity of the genome. For instance, alternative splicing can lead to the creation of proteins with distinct subcellular localizations, enzymatic activities, or binding properties, which are essential for cellular differentiation and adaptation to changing conditions. The significance of alternative splicing is underscored by its widespread occurrence in eukaryotic organisms. It is estimated that more than 90% of human genes undergo alternative splicing, highlighting its critical role in generating proteomic diversity. This mechanism is particularly important in tissues that require a high degree of cellular specialization, such as the brain and immune system. In these contexts, alternative splicing enables the production of tissue-specific isoforms that are tailored to meet the unique functional demands of each cell type. Moreover, dysregulation of alternative splicing has been implicated in various diseases, including cancer, neurological disorders, and developmental abnormalities. Mutations affecting splicing regulatory elements or splicing factors can lead to aberrant splicing patterns, resulting in the production of dysfunctional proteins or the loss of essential isoforms. Understanding the mechanisms and regulation of alternative splicing is therefore crucial for developing therapeutic strategies aimed at correcting these splicing defects. In conclusion, gene expression, particularly through mechanisms like alternative splicing, is a vital process that underpins the complexity and adaptability of life. By allowing a single gene to encode multiple proteins, alternative splicing significantly expands the functional repertoire of the genome, enabling cells to respond to diverse signals and maintain intricate cellular processes. As research continues to unravel the intricacies of alternative splicing, it holds promise for advancing our understanding of cellular biology and developing novel treatments for a range of diseases.
Mechanisms and Regulation of Alternative Splicing
Alternative splicing is a complex and highly regulated process that allows a single gene to produce multiple proteins, thereby significantly expanding the proteomic diversity of an organism. This intricate mechanism is crucial for the fine-tuning of gene expression and is essential for various cellular processes, including development, tissue differentiation, and response to environmental changes. The article delves into the mechanisms and regulation of alternative splicing, exploring three key aspects: the splicing process and key players, regulatory elements and factors, and tissue-specific and developmental splicing. Understanding the splicing process and identifying the key players involved is fundamental to grasping how alternative splicing operates. This includes the roles of spliceosomes, small nuclear ribonucleoproteins (snRNPs), and other essential components that facilitate the removal of introns and the joining of exons. Regulatory elements and factors, such as splicing enhancers and silencers, also play critical roles in determining which exons are included or excluded from the final mRNA transcript. Additionally, tissue-specific and developmental splicing patterns highlight the dynamic nature of alternative splicing, where different cell types and developmental stages exhibit unique splicing profiles. By examining these aspects, we can gain a comprehensive understanding of how alternative splicing is orchestrated and how it contributes to cellular diversity and function. Let us begin by exploring the splicing process and key players, which form the foundational framework for this multifaceted phenomenon.
Splicing Process and Key Players
Alternative splicing, a fundamental process in molecular biology, involves the selective inclusion or exclusion of exons during the splicing of pre-mRNA to generate diverse mature mRNA transcripts from a single gene. This intricate process is crucial for increasing the complexity of the proteome and allowing cells to respond to various environmental and developmental cues. The splicing process itself is highly regulated and involves several key players. At the heart of alternative splicing are the spliceosomes, complex molecular machines composed of small nuclear ribonucleoproteins (snRNPs) and associated proteins. These spliceosomes recognize splice sites on the pre-mRNA and catalyze the removal of introns while joining exons together. The recognition of splice sites is mediated by specific sequences known as splice sites (5' and 3' splice sites) and branch points, which are recognized by snRNAs within the spliceosome. Several key regulatory elements and proteins play pivotal roles in modulating alternative splicing. **Splicing factors**, such as serine/arginine (SR) proteins and heterogeneous nuclear ribonucleoproteins (hnRNPs), bind to specific sequences within the pre-mRNA to either enhance or inhibit the use of particular splice sites. These factors can act as either activators or repressors, depending on their binding sites relative to the splice sites. For instance, SR proteins generally promote splicing by stabilizing the interaction between the pre-mRNA and the spliceosome, while certain hnRNPs can block access to splice sites, thereby inhibiting splicing. Another critical layer of regulation involves **cis-regulatory elements** such as exonic splicing enhancers (ESEs), exonic splicing silencers (ESSs), intronic splicing enhancers (ISEs), and intronic splicing silencers (ISSs). These elements serve as binding platforms for splicing factors, influencing the recruitment of the spliceosome and determining which exons are included or excluded in the final mRNA transcript. Additionally, **post-translational modifications** of splicing factors, such as phosphorylation, can significantly impact their activity and binding affinity, thereby modulating splicing outcomes. For example, the phosphorylation state of SR proteins can alter their ability to interact with other splicing factors and the pre-mRNA, thus influencing alternative splicing patterns. The dynamic interplay between these regulatory elements and proteins ensures that alternative splicing is tightly controlled and responsive to cellular needs. This complexity allows for the generation of a vast array of transcript isoforms from a relatively small number of genes, contributing to the functional diversity of proteins and enabling cells to adapt to changing conditions. In summary, the splicing process is a highly regulated and dynamic mechanism that relies on the coordinated action of spliceosomes, splicing factors, cis-regulatory elements, and post-translational modifications. Understanding these key players and their interactions is essential for elucidating how alternative splicing contributes to cellular function and disease pathogenesis.
Regulatory Elements and Factors
Regulatory elements and factors play a crucial role in the mechanisms and regulation of alternative splicing, a process that allows a single gene to produce multiple proteins. These elements and factors act as the molecular switches that determine which exons are included or excluded from the final mRNA transcript, thereby influencing the diversity of the proteome. **Exonic splicing enhancers (ESEs) and silencers (ESSs)** are key regulatory sequences within exons that recruit splicing factors to either promote or inhibit splice site recognition. For instance, ESEs attract serine/arginine-rich (SR) proteins, which facilitate the assembly of the spliceosome, the complex responsible for catalyzing the splicing reaction. Conversely, ESSs bind to heterogeneous nuclear ribonucleoproteins (hnRNPs) that antagonize SR proteins, thereby preventing exon inclusion. **Intronic splicing enhancers (ISEs) and silencers (ISSs)**, located within introns, also modulate splicing outcomes by interacting with various splicing factors. These intronic elements can either enhance or repress the use of nearby splice sites, adding another layer of complexity to the splicing process. Additionally, **branch point sequences** and **polyprimidine tracts** are essential for the recognition of 3' splice sites by the spliceosome. The precise positioning and sequence of these elements are critical for ensuring accurate and efficient splicing. Splicing factors themselves are proteins that bind to these regulatory elements to orchestrate the splicing process. **Serine/arginine-rich (SR) proteins** and **heterogeneous nuclear ribonucleoproteins (hnRNPs)** are two major classes of splicing factors. SR proteins generally promote exon inclusion by stabilizing the interaction between the pre-mRNA and the spliceosome, while hnRNPs often act as repressors by blocking SR protein binding or recruiting other inhibitory factors. The balance between these opposing forces is finely tuned and can be influenced by various cellular signals, such as phosphorylation events that modify the activity of splicing factors. The regulation of alternative splicing is further complicated by the involvement of **trans-acting factors**, which can be influenced by external signals such as developmental cues, environmental changes, or disease states. For example, certain transcription factors can regulate the expression of splicing factors, thereby indirectly influencing alternative splicing patterns. Moreover, **RNA-binding proteins** like Nova and Fox proteins can bind to specific sequences within pre-mRNAs to modulate their splicing outcomes in a tissue-specific manner. In summary, the intricate interplay between regulatory elements and splicing factors is central to the mechanisms and regulation of alternative splicing. These elements and factors work in concert to ensure that the correct exons are included or excluded from the final mRNA transcript, thereby contributing to the remarkable diversity of the proteome. Understanding these regulatory mechanisms is crucial for elucidating how alternative splicing contributes to cellular function and how its dysregulation can lead to disease.
Tissue-Specific and Developmental Splicing
Alternative splicing, a fundamental process in gene expression, allows a single gene to produce multiple proteins with diverse functions. One of the critical aspects of alternative splicing is its tissue-specific and developmental regulation, which ensures that the right protein isoforms are produced in the appropriate tissues and at the correct stages of development. This precision is crucial for maintaining cellular homeostasis and facilitating the complex processes involved in growth, differentiation, and adaptation. Tissue-specific splicing involves the differential inclusion or exclusion of exons in various tissues, leading to the generation of tissue-specific isoforms. For instance, the muscle-specific isoform of the troponin T gene includes exons that are skipped in other tissues, enabling the production of a protein tailored for muscle function. This specificity is achieved through a combination of cis-regulatory elements within the pre-mRNA and trans-acting factors such as splicing enhancers and silencers that bind to these elements. These regulatory elements can be recognized by tissue-specific splicing factors, which either promote or inhibit the use of particular splice sites. Developmental splicing, on the other hand, involves changes in splicing patterns as an organism develops from embryonic stages to adulthood. During embryogenesis, for example, certain genes undergo alternative splicing to produce isoforms that are essential for early developmental processes. As the organism matures, these splicing patterns can shift to accommodate the needs of adult tissues. The neural cell adhesion molecule (NCAM) gene is a classic example where different isoforms are produced at different developmental stages, influencing cell-cell interactions and neural development. The regulation of tissue-specific and developmental splicing is tightly controlled by a complex interplay of factors. Splicing factors such as SR proteins and hnRNPs play pivotal roles by binding to specific sequences within the pre-mRNA and influencing the assembly of the spliceosome. Additionally, epigenetic modifications like DNA methylation and histone modifications can impact chromatin structure, thereby affecting the accessibility of splicing regulatory elements to trans-acting factors. Moreover, recent studies have highlighted the role of non-coding RNAs, particularly microRNAs and long non-coding RNAs, in modulating splicing outcomes. These RNAs can bind to splicing factors or directly to pre-mRNA, thereby influencing the inclusion or exclusion of exons in a tissue-specific or developmentally regulated manner. In summary, tissue-specific and developmental splicing are intricate processes that ensure the precise production of protein isoforms necessary for the proper functioning of different tissues and developmental stages. The regulation of these processes involves a sophisticated network of cis-regulatory elements, trans-acting factors, epigenetic modifications, and non-coding RNAs, underscoring the complexity and importance of alternative splicing in maintaining cellular diversity and organismal health. Understanding these mechanisms not only deepens our insight into gene regulation but also opens avenues for therapeutic interventions in diseases where splicing dysregulation plays a critical role.
Biological and Clinical Implications of Alternative Splicing
Alternative splicing, a fundamental process in molecular biology, significantly expands the functional diversity of the genome by generating multiple protein isoforms from a single gene. This complex mechanism has profound implications both biologically and clinically, influencing various aspects of cellular function and disease pathology. The diversity of protein isoforms resulting from alternative splicing allows cells to fine-tune their responses to different environmental cues, thereby enhancing cellular adaptability and complexity. However, dysregulation of alternative splicing can lead to the production of aberrant protein isoforms that contribute to disease pathogenesis, particularly in conditions such as cancer, neurological disorders, and genetic diseases. Understanding these mechanisms also opens up therapeutic potential, as targeting specific splicing events could offer novel avenues for treatment. This article delves into these critical aspects, starting with an exploration of the **Diversity of Protein Isoforms**, which underscores the intricate and multifaceted nature of alternative splicing and its role in shaping cellular function.
Diversity of Protein Isoforms
The diversity of protein isoforms, generated through the process of alternative splicing, is a cornerstone of cellular complexity and adaptability. Alternative splicing allows a single gene to produce multiple distinct proteins, each with potentially unique functions, subcellular localizations, and interactions. This phenomenon is crucial for the fine-tuning of cellular processes, enabling cells to respond to various environmental cues and developmental signals. For instance, alternative splicing can introduce or remove specific protein domains, alter binding sites for other proteins or ligands, and even change the overall structure of the protein. This versatility is particularly evident in the nervous system, where alternative splicing contributes to the vast array of neuronal functions and synaptic plasticity, essential for learning and memory. In terms of biological implications, the diversity of protein isoforms enhances the functional repertoire of cells. For example, in muscle cells, different isoforms of the troponin T protein are generated through alternative splicing, each tailored to the specific needs of different muscle types (skeletal, cardiac, or smooth muscle). Similarly, in immune cells, alternative splicing of genes involved in the immune response allows for a more nuanced and context-dependent immune reaction. This adaptability is vital for the immune system's ability to recognize and respond to a wide range of pathogens. Clinically, the implications of protein isoform diversity are profound. Misregulation of alternative splicing has been linked to numerous diseases, including cancer, neurological disorders such as Alzheimer's and Parkinson's diseases, and muscular dystrophies. For instance, aberrant splicing patterns can lead to the production of oncogenic protein isoforms that promote tumor growth and metastasis. Conversely, therapeutic strategies targeting specific splicing events are being explored as potential treatments for these diseases. RNA-based therapies, such as antisense oligonucleotides and small molecule splicing modulators, aim to correct splicing defects or modulate the expression of beneficial protein isoforms. Moreover, understanding the diversity of protein isoforms has significant implications for personalized medicine. Genetic variations that affect splicing can influence an individual's susceptibility to certain diseases and their response to treatments. By analyzing these variations and their impact on splicing patterns, clinicians can develop more tailored therapeutic approaches. For example, in cancer treatment, identifying specific splicing variants associated with drug resistance could help in designing targeted therapies that overcome this resistance. In conclusion, the diversity of protein isoforms resulting from alternative splicing is a critical aspect of cellular biology with far-reaching biological and clinical implications. It underscores the intricate complexity of gene expression and highlights the potential for therapeutic interventions that target specific splicing events. As research continues to unravel the mechanisms and consequences of alternative splicing, it promises to reveal new avenues for understanding disease pathogenesis and developing innovative treatments.
Role in Disease Pathogenesis
Alternative splicing plays a pivotal role in disease pathogenesis, significantly influencing the complexity and diversity of the proteome. This process, where a single gene can give rise to multiple protein isoforms through differential exon inclusion or exclusion, is crucial for cellular function and regulation. However, aberrant alternative splicing can lead to the production of dysfunctional or toxic protein variants that contribute to various diseases. In cancer, for instance, alternative splicing can result in the generation of oncogenic isoforms that promote tumor growth and metastasis. For example, the splicing factor SRSF1 is often overexpressed in cancer cells, leading to the production of pro-oncogenic isoforms of genes involved in cell proliferation and survival. Similarly, in neurological disorders such as amyotrophic lateral sclerosis (ALS) and frontotemporal dementia (FTD), mutations in genes like TDP-43 and FUS disrupt normal splicing patterns, resulting in the accumulation of toxic RNA species that are harmful to neurons. In autoimmune diseases like systemic lupus erythematosus (SLE), aberrant alternative splicing can lead to the production of autoantigens that trigger immune responses. The splicing factor hnRNP A1, for example, is known to be involved in the regulation of autoantigen expression in SLE patients. Furthermore, in cardiovascular diseases such as atherosclerosis, alternative splicing of genes involved in lipid metabolism and inflammation can contribute to plaque formation and vascular dysfunction. The clinical implications of these findings are profound. Understanding the role of alternative splicing in disease pathogenesis opens up new avenues for therapeutic intervention. Targeting specific splicing factors or modulating splicing patterns could provide novel treatments for a wide range of diseases. For instance, small molecule inhibitors that selectively target oncogenic splicing factors are being explored as potential cancer therapies. Additionally, RNA-based therapies such as antisense oligonucleotides and RNA interference (RNAi) can be designed to correct aberrant splicing patterns, offering hope for treating genetic disorders caused by splicing mutations. Moreover, the study of alternative splicing has highlighted the importance of considering the complexity of gene expression when interpreting genomic data. This is particularly relevant in personalized medicine, where understanding an individual's unique splicing profile could help tailor treatment strategies to their specific genetic and molecular context. In conclusion, the role of alternative splicing in disease pathogenesis underscores its significance as a critical regulatory mechanism that, when dysregulated, can have far-reaching consequences for human health. Elucidating these mechanisms not only deepens our understanding of disease biology but also paves the way for innovative therapeutic approaches aimed at restoring normal splicing patterns and improving patient outcomes.
Therapeutic Potential and Applications
Alternative splicing, a fundamental process in gene expression, holds significant therapeutic potential and diverse applications, particularly in the realm of biological and clinical research. This mechanism allows a single gene to produce multiple proteins, each with distinct functions, thereby enhancing cellular versatility and complexity. The therapeutic implications of alternative splicing are multifaceted. For instance, understanding the splicing patterns associated with specific diseases can lead to the development of targeted therapies. In cancer, certain splice variants are known to promote tumor progression or resistance to treatments. By identifying and inhibiting these harmful variants, researchers can design more effective cancer therapies. Similarly, in neurological disorders such as spinal muscular atrophy (SMA), therapeutic strategies like antisense oligonucleotides can be used to modulate splicing patterns and restore functional protein expression. Moreover, alternative splicing plays a crucial role in drug development. Many drugs target proteins that are produced through alternative splicing pathways. For example, certain splice variants of the androgen receptor are implicated in prostate cancer, making them potential targets for therapeutic intervention. Additionally, the study of alternative splicing can provide insights into drug resistance mechanisms. By analyzing how splicing patterns change in response to drug treatment, scientists can develop strategies to overcome resistance and improve treatment efficacy. From a clinical perspective, the analysis of alternative splicing can serve as a valuable diagnostic tool. Specific splice variants may be associated with particular disease states or prognostic outcomes, allowing for more precise diagnosis and personalized treatment plans. For instance, in cardiovascular diseases, certain splice variants of genes involved in cardiac function can predict susceptibility to heart failure or arrhythmias. This information can guide clinicians in making informed decisions about patient care and management. Furthermore, advances in RNA sequencing technologies have enabled the comprehensive analysis of alternative splicing across various tissues and conditions. This has opened up new avenues for biomarker discovery and the development of RNA-based therapies. For example, RNA interference (RNAi) and CRISPR-Cas systems can be designed to specifically target and modulate disease-associated splice variants, offering novel therapeutic approaches. In summary, the therapeutic potential and applications of alternative splicing are vast and promising. By unraveling the complexities of this process, scientists can develop targeted therapies, improve drug efficacy, enhance diagnostic capabilities, and ultimately contribute to better patient outcomes. As research continues to elucidate the intricate mechanisms of alternative splicing, its clinical implications will become increasingly clear, paving the way for innovative treatments and improved healthcare strategies.