Why Does Ionization Energy Increase Across A Period
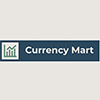
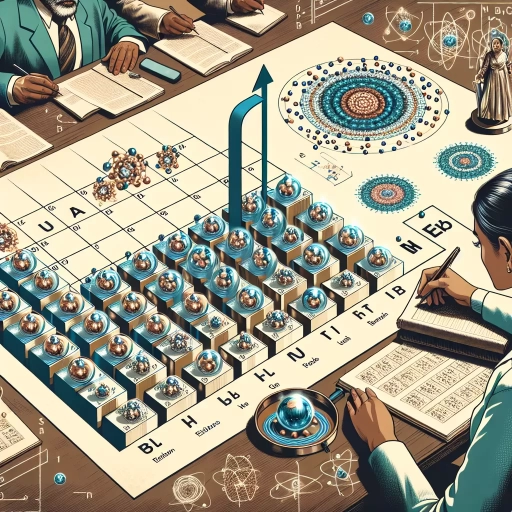
Ionization energy, the energy required to remove an electron from a neutral atom, is a fundamental concept in chemistry that exhibits intriguing trends across the periodic table. One of the most notable trends is the increase in ionization energy as you move from left to right across a period. This phenomenon can be attributed to several key factors, each playing a crucial role in understanding the underlying chemistry. Firstly, the decrease in atomic radius as you traverse a period significantly impacts ionization energy, as smaller atoms hold their electrons more tightly. Additionally, changes in electron configuration and the effects of shielding also contribute to this trend, influencing how easily an electron can be removed. Lastly, periodic trends and electron affinity provide a broader context, highlighting how the tendency of an atom to attract electrons affects its ionization energy. In this article, we will delve into these supporting ideas, starting with the critical relationship between atomic radius and its impact on ionization energy. By exploring these factors, we will gain a deeper understanding of why ionization energy increases across a period.
Atomic Radius and Its Impact on Ionization Energy
Atomic radius, a fundamental concept in chemistry, plays a crucial role in understanding the behavior of elements and their interactions. As we traverse the periodic table, particularly across a period, the atomic radius undergoes significant changes that have profound implications on various chemical properties. One such property is ionization energy, which is the energy required to remove an electron from an atom. The decrease in atomic radius across a period is a key factor influencing ionization energy. This reduction is primarily due to the increased effective nuclear charge, where the addition of protons to the nucleus enhances its pull on electrons. Consequently, this stronger electrostatic attraction between the nucleus and electrons makes it more difficult to remove an electron, thereby increasing the ionization energy. Understanding these relationships is essential for grasping how atomic radius impacts ionization energy, and this article will delve into these concepts to provide a comprehensive insight into their interplay. By exploring the decrease in atomic radius across a period, the increased effective nuclear charge, and the stronger electrostatic attraction between the nucleus and electrons, we will uncover the intricate mechanisms behind the impact of atomic radius on ionization energy.
Decrease in Atomic Radius Across a Period
As we traverse a period in the periodic table, a notable trend emerges: the atomic radius decreases. This phenomenon is crucial for understanding various chemical properties, including ionization energy. The decrease in atomic radius across a period can be attributed to the increasing effective nuclear charge and the addition of electrons to the same principal energy level. When moving from left to right within a period, each successive element has one more proton in its nucleus, which enhances the nuclear charge. This increased positive charge pulls the electrons closer to the nucleus, resulting in a smaller atomic radius. Additionally, although electrons are added to the same principal energy level (or shell), they do not shield each other effectively due to their similar energies and spatial distributions. Consequently, the effective nuclear charge experienced by each electron increases, drawing them closer to the nucleus and reducing the overall size of the atom. This contraction in atomic radius has significant implications for ionization energy. As atoms become smaller, the outermost electrons are held more tightly by the nucleus due to the increased effective nuclear charge. This tighter binding makes it more difficult to remove an electron from the atom, thereby increasing the ionization energy. The relationship between atomic radius and ionization energy is thus inversely related: as atomic radius decreases across a period, ionization energy increases. This trend is a fundamental principle in understanding why elements exhibit varying reactivities and why certain elements are more prone to losing or gaining electrons than others. In summary, the decrease in atomic radius across a period is driven by the increasing effective nuclear charge and the inefficient shielding of electrons within the same principal energy level. This reduction in size directly influences ionization energy, making it more energetically costly to remove an electron from smaller atoms. Understanding this trend is essential for predicting chemical behavior and explaining why ionization energy increases as we move across a period in the periodic table.
Increased Effective Nuclear Charge
As we delve into the intricacies of atomic radius and its impact on ionization energy, a crucial concept that emerges is the increased effective nuclear charge. This phenomenon plays a pivotal role in understanding why ionization energy tends to rise as we move across a period in the periodic table. Effective nuclear charge refers to the net positive charge experienced by an electron in an atom, which is influenced by the number of protons in the nucleus and the shielding effect of inner electrons. As we traverse a period from left to right, the atomic number increases, meaning there are more protons in the nucleus. This increase in protons enhances the effective nuclear charge because each additional proton adds to the overall positive charge. However, the key factor here is not just the increase in protons but also the lack of significant increase in the number of electron shells. Across a period, electrons are added to the same principal energy level, which means they do not experience substantial shielding from inner electrons. Consequently, each electron added to the outermost shell feels a stronger pull from the nucleus due to this increased effective nuclear charge. This stronger attraction makes it more difficult to remove an electron from the atom, thereby increasing the ionization energy. The impact of increased effective nuclear charge is further compounded by the fact that electrons in the same principal energy level do not shield each other very effectively. As a result, each subsequent electron added across a period experiences a slightly higher effective nuclear charge than the previous one, leading to a steady increase in ionization energy. This trend is evident when comparing elements within the same period; for instance, ionization energy generally increases from sodium to neon as we move from left to right in the second period of the periodic table. In summary, the increase in effective nuclear charge across a period is a direct consequence of the rising atomic number and the minimal shielding effect from electrons in the same principal energy level. This heightened effective nuclear charge results in a stronger electrostatic attraction between the nucleus and outer electrons, making it increasingly difficult to remove an electron and thus leading to higher ionization energies. Understanding this concept is essential for grasping why ionization energy increases as we traverse a period in the periodic table, highlighting the intricate interplay between atomic structure and chemical properties.
Stronger Electrostatic Attraction Between Nucleus and Electrons
As atomic radius decreases across a period, the stronger electrostatic attraction between the nucleus and electrons plays a pivotal role in understanding why ionization energy increases. This phenomenon is rooted in the fundamental principles of atomic structure and electrostatic forces. When an atom's radius diminishes, the distance between the nucleus and the outermost electrons decreases, leading to an enhanced electrostatic pull. This increased attraction is due to Coulomb's Law, which states that the force between two charged particles is inversely proportional to the square of the distance between them. Consequently, as the atomic radius shrinks, the electrostatic force holding electrons to the nucleus becomes stronger. This stronger attraction has several implications for ionization energy. Ionization energy is the amount of energy required to remove an electron from an atom in its ground state. With a smaller atomic radius, the outermost electrons are held more tightly by the nucleus, making it more difficult to remove them. Therefore, more energy is needed to overcome this increased electrostatic attraction, resulting in higher ionization energies as you move across a period. Additionally, the effective nuclear charge—the net positive charge experienced by an electron—increases as you move across a period due to the addition of protons in the nucleus and the inefficient shielding by inner electrons. This rise in effective nuclear charge further exacerbates the electrostatic attraction, contributing to the observed trend of increasing ionization energy. The interplay between atomic radius and electrostatic attraction also influences other atomic properties. For instance, smaller atoms tend to have higher electronegativities because their electrons are more tightly bound, making them more resistant to losing or gaining electrons. This resistance is a direct consequence of the stronger electrostatic forces at play. Moreover, the increased stability of smaller atoms due to their stronger nuclear-electron attraction can affect chemical reactivity and bonding patterns, as these atoms are less likely to participate in reactions that involve electron transfer. In summary, the decrease in atomic radius across a period leads to a significant increase in the electrostatic attraction between the nucleus and electrons. This enhanced attraction is a key factor in understanding why ionization energy rises as you move from left to right across the periodic table. The combination of reduced atomic size and increased effective nuclear charge creates an environment where electrons are more tightly bound, necessitating higher energies for their removal and thus explaining the trend of increasing ionization energy across a period.
Electron Configuration and Shielding Effects
Electron configuration and shielding effects are fundamental concepts in chemistry that underpin our understanding of atomic structure and its influence on chemical properties. The arrangement of electrons within an atom, described by electron configuration, dictates how atoms interact with each other. However, this arrangement is not static; it changes significantly across a period due to the addition of electrons to the same principal energy level, leading to variations in atomic radius and ionization energy. Additionally, the shielding effect, which reduces the attractive force between the nucleus and outer electrons, is compromised by poor shielding from d and f orbitals. This inefficiency in shielding contributes to the overall electron configuration and its impact on atomic properties. Furthermore, the increased penetration of s orbitals towards the nucleus enhances the effective nuclear charge experienced by these electrons, further influencing atomic radius and ionization energy. Understanding these dynamics is crucial for grasping how atomic radius changes and its subsequent impact on ionization energy, making electron configuration and shielding effects essential topics in chemistry.
Changes in Electron Configuration Across a Period
As we traverse a period in the periodic table, significant changes occur in the electron configuration of elements, which have profound implications for their chemical properties and behavior. At the beginning of a period, the outermost energy level starts to fill with electrons in the s-orbitals, typically resulting in one or two electrons in the s-orbital for the first two elements. As we move across the period, these s-orbitals are filled, and then the p-orbitals begin to fill. The p-orbitals can accommodate up to six electrons, leading to a gradual increase in the number of valence electrons as we progress. This increase in valence electrons is accompanied by a decrease in atomic radius due to the ineffective shielding of nuclear charge by additional electrons in the same principal energy level. The electrons in the same shell do not effectively shield one another from the increasing nuclear charge, leading to a stronger attraction between the nucleus and the outermost electrons. Consequently, this results in higher ionization energies as it becomes more difficult to remove an electron from an atom with a stronger nuclear pull. Furthermore, the electron configuration changes also influence the electron-electron repulsions within the valence shell. As more electrons occupy the p-orbitals, these repulsions increase, contributing to higher ionization energies. Additionally, elements towards the end of a period often exhibit full or nearly full outer energy levels, which are particularly stable configurations. Removing an electron from such a stable configuration requires more energy, further contributing to the trend of increasing ionization energy across a period. The interplay between these factors—increasing nuclear charge, ineffective shielding, and electron-electron repulsions—culminates in a systematic rise in ionization energy as we move from left to right across a period. This trend is crucial for understanding various chemical phenomena, including reactivity patterns and the formation of ions. The changes in electron configuration not only dictate how easily an atom can lose an electron but also underpin many of the periodic trends that are fundamental to chemistry. Thus, grasping these changes is essential for a comprehensive understanding of why ionization energy increases across a period.
Decrease in Shielding Effect Due to Poor Shielding by d and f Orbitals
The decrease in shielding effect due to poor shielding by d and f orbitals is a crucial concept in understanding the trends in ionization energy across a period. In atomic physics, shielding refers to the reduction in the attractive force between the nucleus and an electron due to the presence of inner electrons. Effective shielding occurs when inner electrons completely fill s and p orbitals, which are spherical in shape and thus provide uniform shielding around the nucleus. However, d and f orbitals have different shapes and orientations that lead to less effective shielding. D orbitals, for instance, are dumbbell-shaped and have four lobes, while f orbitals have six lobes with complex shapes. These shapes result in less effective overlap with the nucleus compared to s and p orbitals. Consequently, electrons in d and f orbitals do not shield outer electrons as efficiently, leading to a weaker reduction in the nuclear charge experienced by these outer electrons. This inefficiency means that the effective nuclear charge felt by outer electrons increases more significantly as you move across a period, especially when transitioning from s and p blocks to d and f blocks. This phenomenon has significant implications for ionization energy. Ionization energy is the energy required to remove an electron from an atom. As you move across a period, the poor shielding by d and f orbitals results in a higher effective nuclear charge on the outermost electrons. This increased nuclear attraction makes it more difficult to remove an electron, thereby increasing the ionization energy. The trend is particularly pronounced in transition metals and lanthanides where d and f orbitals are being filled, respectively. Here, despite the addition of electrons to these less shielding orbitals, the overall effect is an increase in ionization energy due to the inadequate shielding of the nuclear charge. In summary, the poor shielding effect of d and f orbitals plays a pivotal role in explaining why ionization energy increases across a period. The unique shapes of these orbitals lead to less effective shielding, resulting in a higher effective nuclear charge on outer electrons. This increased nuclear attraction necessitates more energy to remove an electron, thus contributing to the observed trend of increasing ionization energy as one moves from left to right across the periodic table. Understanding this concept is essential for grasping the underlying principles of electron configuration and its impact on atomic properties.
Increased Penetration of s Orbitals Towards the Nucleus
As we delve into the intricacies of electron configuration and shielding effects, it becomes crucial to understand the increased penetration of s orbitals towards the nucleus. This phenomenon plays a pivotal role in explaining why ionization energy increases across a period in the periodic table. In atomic physics, the penetration of an orbital refers to how deeply it extends into the region close to the nucleus. s orbitals, due to their spherical symmetry, have a higher probability density near the nucleus compared to p, d, or f orbitals. This increased penetration means that electrons in s orbitals are more likely to be found closer to the nucleus, where they experience a stronger attractive force from the positively charged protons. As we move across a period from left to right, the atomic number increases, which means there are more protons in the nucleus. This increase in nuclear charge enhances the electrostatic attraction between the nucleus and electrons. However, because s orbitals penetrate more effectively than other types of orbitals, electrons in these orbitals are particularly affected by this increased nuclear charge. The result is that as we traverse a period, the effective nuclear charge felt by electrons in s orbitals increases significantly. This heightened attraction makes it more difficult to remove an electron from an atom, thereby increasing its ionization energy. Additionally, the shielding effect—where inner electrons partially shield outer electrons from the full force of the nuclear charge—becomes less effective as we move across a period due to the poor shielding ability of s electrons compared to other orbitals. In summary, the increased penetration of s orbitals towards the nucleus is a key factor in understanding why ionization energy rises across a period. The stronger nuclear attraction experienced by these penetrating s electrons, coupled with reduced shielding effects, contributes significantly to this trend. This fundamental concept underscores the importance of orbital penetration in explaining various periodic trends and highlights how electron configuration and shielding effects interplay to shape atomic properties.
Periodic Trends and Electron Affinity
Understanding periodic trends is crucial for grasping the fundamental properties of elements, and electron affinity is a key aspect of these trends. Electron affinity, the energy change when an electron is added to a neutral atom, varies systematically across the periodic table. This article delves into the intricate relationships that govern electron affinity, exploring three pivotal concepts: the relationship between ionization energy and electron affinity, the general increase in electron affinity across a period, and the impact of half-filled and fully filled subshells on stability. By examining these factors, we can better comprehend why certain elements exhibit higher or lower electron affinities. Additionally, understanding these trends will naturally transition us to consider atomic radius and its impact on ionization energy, highlighting the interconnected nature of these atomic properties. This comprehensive approach will provide a clear and insightful look into the periodic trends that shape the behavior of elements in the periodic table.
Relationship Between Ionization Energy and Electron Affinity
The relationship between ionization energy and electron affinity is a crucial aspect of understanding periodic trends in chemistry. Ionization energy, the energy required to remove an electron from a neutral atom, generally increases across a period due to the increasing effective nuclear charge and decreasing atomic radius. Conversely, electron affinity, which is the energy released when an electron is added to a neutral atom, exhibits a more complex trend but often shows a similar increase across a period for the same reasons. As you move from left to right across a period, the number of protons in the nucleus increases, leading to a stronger attraction between the nucleus and the electrons. This increased nuclear charge pulls the electrons closer to the nucleus, reducing the atomic radius. Consequently, it becomes more difficult to remove an electron from the atom, resulting in higher ionization energies. Similarly, adding an electron to an atom with a higher effective nuclear charge is more favorable because the electron is more strongly attracted to the nucleus, which can lead to higher electron affinities. However, electron affinity does not always follow this straightforward trend. For instance, elements like nitrogen and oxygen have lower electron affinities compared to their neighbors due to the stability of their half-filled or full subshells. Adding an electron to these atoms would disrupt this stability, making it less energetically favorable. Despite these exceptions, the overall trend of increasing ionization energy and generally increasing electron affinity across a period is driven by the same fundamental principles of nuclear charge and atomic size. Understanding these trends is essential for predicting chemical reactivity and stability. For example, elements with high ionization energies and electron affinities tend to be less reactive because they are less likely to lose or gain electrons easily. This knowledge helps chemists predict how different elements will behave in various chemical reactions and environments. In summary, the relationship between ionization energy and electron affinity is deeply intertwined with periodic trends. Both properties are influenced by the same underlying factors—nuclear charge and atomic radius—which generally lead to an increase in these energies across a period. While there are exceptions due to specific electron configurations, grasping these trends provides valuable insights into the chemical behavior of elements and their reactivity patterns.
General Increase in Electron Affinity Across a Period
As we traverse a period from left to right in the periodic table, a notable trend emerges in electron affinity, which is the energy change associated with the addition of an electron to a neutral atom. Electron affinity generally increases across a period due to several key factors. Firstly, the atomic radius decreases as we move from left to right within a period. This reduction in size means that the outermost electrons are closer to the nucleus, resulting in a stronger electrostatic attraction between the nucleus and the incoming electron. Consequently, it becomes more energetically favorable for an atom to accept an additional electron, leading to an increase in electron affinity. Secondly, the effective nuclear charge increases across a period. As we add more protons to the nucleus while moving across a period, the positive charge of the nucleus increases. Although the electrons in the same principal energy level do not shield each other effectively, the increased nuclear charge pulls the electrons closer, enhancing the atom's ability to attract an additional electron. However, there are exceptions to this trend. For instance, elements in Group 2 (alkaline earth metals) and Group 17 (halogens) often exhibit lower electron affinities compared to their neighbors. In Group 2 elements, adding an electron would result in a full s-orbital, which is less stable than a half-filled orbital due to electron-electron repulsion. For Group 17 elements, adding an electron would complete their outermost energy level, but this process is less favorable because it involves adding an electron to a region already densely populated with electrons. Despite these exceptions, the overall trend of increasing electron affinity across a period remains a fundamental principle in understanding periodic trends. This trend is crucial for predicting chemical behavior and reactivity patterns among elements. For example, it helps explain why certain elements are more likely to form anions and participate in ionic bonding. In summary, the general increase in electron affinity across a period is driven by decreasing atomic radius and increasing effective nuclear charge. These factors enhance the electrostatic attraction between the nucleus and incoming electrons, making it more energetically favorable for atoms to accept additional electrons as we move from left to right across the periodic table. Understanding this trend provides valuable insights into chemical properties and reactivity, underscoring its importance in the broader context of periodic trends and ionization energy.
Impact of Half-Filled and Fully Filled Subshells on Stability
The stability of atoms is significantly influenced by the filling of subshells, particularly when they are half-filled or fully filled. This phenomenon is a crucial aspect of understanding periodic trends, including electron affinity and ionization energy. When subshells are half-filled or fully filled, they achieve a particularly stable electronic configuration due to the symmetrical distribution of electrons. In a half-filled subshell, each orbital contains one electron, maximizing the number of unpaired electrons and minimizing electron-electron repulsion. This results in a lower energy state and enhanced stability. For instance, chromium (Cr) and manganese (Mn) exhibit half-filled subshells in their ground states, leading to their notable stability and lower ionization energies compared to neighboring elements. Similarly, fully filled subshells also contribute to atomic stability. When all orbitals in a subshell are occupied by electrons, the subshell is completely filled, leading to a highly stable configuration. This is evident in the noble gases, such as neon (Ne) and argon (Ar), which have fully filled outer energy levels. These elements are chemically inert due to their stable electronic configurations, making it difficult to remove or add electrons. The stability associated with fully filled subshells is a key reason why noble gases have high ionization energies and low electron affinities; they are reluctant to either lose or gain electrons. The impact of these stable configurations on periodic trends is profound. Across a period, as the atomic number increases, electrons are added to the same principal energy level but to different subshells. The tendency to achieve half-filled or fully filled subshells can lead to variations in ionization energy and electron affinity. For example, elements like boron (B) and aluminum (Al) exhibit lower ionization energies due to their desire to achieve a more stable configuration by losing electrons. Conversely, elements nearing the end of a period, such as fluorine (F) and neon (Ne), have high ionization energies because their outer subshells are nearly or completely filled, making it energetically unfavorable to remove an electron. In summary, the stability imparted by half-filled and fully filled subshells plays a pivotal role in understanding periodic trends. These configurations influence ionization energy and electron affinity, making certain elements more or less reactive based on their electronic structures. The quest for achieving these stable configurations drives many of the chemical properties observed across the periodic table, highlighting the fundamental importance of subshell filling in atomic stability.