When Does A Conductor Produce A Magnetic Field
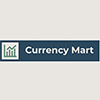
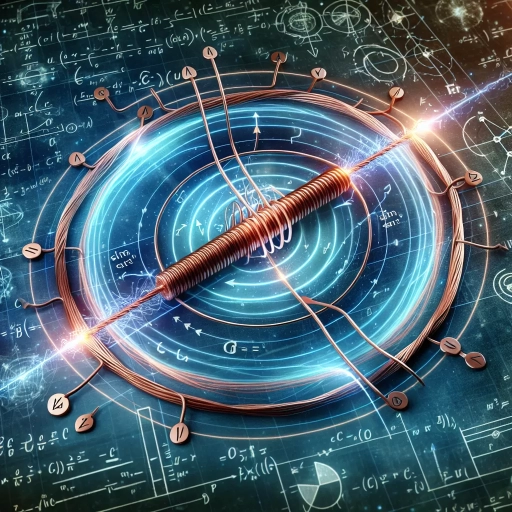
The phenomenon of a conductor producing a magnetic field is a fundamental aspect of electromagnetism, a branch of physics that underpins many modern technologies. At its core, this phenomenon involves the interaction between electric currents and magnetic fields, which is crucial for understanding various practical applications in engineering, electronics, and even everyday life. To delve into this topic, it is essential to first grasp the basics of electromagnetism, which will provide the foundational knowledge necessary for comprehending how and when a conductor generates a magnetic field. This article will explore three key areas: **Understanding the Basics of Electromagnetism**, which lays out the theoretical groundwork; **Conditions for a Conductor to Produce a Magnetic Field**, detailing the specific circumstances under which this occurs; and **Practical Applications and Observations**, highlighting real-world examples and implications. By starting with an understanding of the fundamental principles, we can then transition seamlessly into the conditions and practical aspects, providing a comprehensive overview of this intriguing phenomenon. Let us begin by **Understanding the Basics of Electromagnetism**.
Understanding the Basics of Electromagnetism
Electromagnetism, a fundamental force of nature, underpins many of the technological advancements and natural phenomena we encounter daily. To grasp the intricacies of this force, it is essential to delve into its core components. This article aims to provide a comprehensive overview of the basics of electromagnetism, starting with a clear **Definition of Electromagnetism** that clarifies its role in the physical world. We will then explore the **Role of Electric Current in Magnetism**, highlighting how the flow of electric charge generates magnetic fields and vice versa. Additionally, we will delve into the **Historical Context and Key Discoveries** that have shaped our understanding of electromagnetism, from the early observations of magnetism to the groundbreaking work of scientists like Maxwell and Faraday. By understanding these foundational aspects, readers will gain a solid foundation in the principles of electromagnetism, enabling them to appreciate its significance and applications in modern science and technology. This journey into the heart of electromagnetism will equip you with the knowledge necessary to appreciate its profound impact on our world, leading you to a deeper **Understanding the Basics of Electromagnetism**.
Definition of Electromagnetism
Electromagnetism is a fundamental physical phenomenon that describes the interaction between electrically charged particles and the electromagnetic force, one of the four fundamental forces of nature. It encompasses both electricity and magnetism, illustrating how these seemingly distinct forces are, in fact, different manifestations of the same underlying physical principle. At its core, electromagnetism involves the generation of magnetic fields by moving electric charges or changing electric fields. This concept is encapsulated in Maxwell's equations, a set of four equations formulated by James Clerk Maxwell that unify the previously separate theories of electricity and magnetism into a single, coherent theory. The essence of electromagnetism lies in the interplay between electric currents and magnetic fields. When an electric current flows through a conductor, such as a wire, it generates a magnetic field around the conductor. This is known as the Biot-Savart law, which quantitatively describes how the magnetic field strength depends on the current, the length of the conductor, and the distance from the conductor. Conversely, a changing magnetic field can induce an electric field, a phenomenon described by Faraday's law of induction. This reciprocal relationship highlights the dynamic and interconnected nature of electromagnetism. Understanding electromagnetism is crucial for explaining a wide range of phenomena, from the operation of simple devices like motors and generators to complex technologies such as radio communication and medical imaging techniques like MRI. In everyday life, electromagnetism is evident in the functioning of household appliances, automotive systems, and even the Earth's own magnetic field, which protects life on our planet from harmful solar radiation. In the context of conductors producing magnetic fields, it is essential to recognize that any movement of charge—whether it be through a wire or within a material—results in the generation of a magnetic field. This principle underpins many technological applications, including electromagnetic induction in transformers and the operation of electromagnetic coils in devices such as speakers and microphones. By grasping the fundamental principles of electromagnetism, one can better appreciate the intricate mechanisms behind these technologies and the broader implications for our understanding of the physical world. Ultimately, electromagnetism serves as a cornerstone of modern physics, bridging the gap between classical mechanics and quantum mechanics while enabling countless innovations that shape our daily lives.
Role of Electric Current in Magnetism
The role of electric current in magnetism is a fundamental concept within the realm of electromagnetism, highlighting the intricate relationship between electricity and magnetism. When an electric current flows through a conductor, it generates a magnetic field around it. This phenomenon is encapsulated by Ampère's law, which states that the magnetic field produced by a current-carrying conductor is directly proportional to the magnitude of the current and inversely proportional to the distance from the conductor. The direction of this magnetic field can be determined using the right-hand rule, where if you point your thumb in the direction of the current flow, your fingers will curl in the direction of the magnetic field lines. This principle underpins various technological applications, including solenoids and electromagnets. In a solenoid, for instance, when an electric current passes through its coils, it creates a strong magnetic field that can be used to perform tasks such as lifting heavy objects or controlling the flow of fluids in industrial processes. Electromagnets, similarly, rely on electric current to produce a magnetic field that can be turned on and off at will, making them indispensable in devices like motors, generators, and magnetic resonance imaging (MRI) machines. The production of a magnetic field by an electric current also explains why conductors exhibit certain behaviors when placed within existing magnetic fields. For example, when a conductor moves through a magnetic field, it experiences an electromotive force (EMF) due to Faraday's law of induction. This EMF can cause the conductor to generate its own electric current, a principle exploited in generators and transformers. Understanding the role of electric current in magnetism is crucial for designing and optimizing electrical systems. It allows engineers to predict and control the interactions between currents and magnetic fields, ensuring efficient operation and safety in electrical devices. Moreover, this knowledge has led to significant advancements in fields such as telecommunications, where the manipulation of electromagnetic waves is essential for signal transmission. In summary, the interplay between electric current and magnetism is a cornerstone of electromagnetism. The generation of a magnetic field by an electric current is not only a theoretical concept but also a practical foundation for numerous technological innovations. As our understanding of this relationship continues to evolve, so too do the possibilities for harnessing and manipulating electromagnetic forces to drive technological progress.
Historical Context and Key Discoveries
Understanding the basics of electromagnetism is deeply rooted in its historical context and key discoveries. The journey to comprehend how conductors produce magnetic fields began with the early observations of magnetism by ancient Greeks, who noted the attractive properties of lodestone. However, it wasn't until the 19th century that significant breakthroughs were made. One pivotal figure was Hans Christian Ørsted, who in 1820 discovered that an electric current generates a magnetic field. This finding was a cornerstone in the development of electromagnetism and led to further investigations by scientists such as André-Marie Ampère, who formulated Ampère's law, which relates the magnetic field around a closed loop to the electric current passing through it. Another crucial discovery was made by Michael Faraday, who introduced the concept of electromagnetic induction in 1831. Faraday's experiments showed that a changing magnetic field induces an electric field, and vice versa, laying the groundwork for understanding how conductors interact with magnetic fields. James Clerk Maxwell later unified these findings into a set of equations known as Maxwell's equations, which are fundamental to modern electromagnetism. Maxwell's work not only predicted the existence of electromagnetic waves but also provided a comprehensive framework for understanding how electric and magnetic fields interact. The discovery of the electron by J.J. Thomson in 1897 further elucidated the nature of electric currents and their role in producing magnetic fields. Thomson's work demonstrated that electric currents are composed of moving charges, which inherently generate magnetic fields according to the principles established by Ørsted and Ampère. The subsequent development of quantum mechanics by physicists like Niels Bohr and Erwin Schrödinger added another layer of understanding, explaining how electrons in conductors behave and contribute to the overall magnetic field. In contemporary times, our understanding of how conductors produce magnetic fields is refined through advanced materials science and technological applications. For instance, superconductors, discovered by Heike Kamerlingh Onnes in 1911, exhibit zero electrical resistance and can produce powerful magnetic fields when cooled to extremely low temperatures. These advancements have led to innovations in fields such as medical imaging (MRI), high-energy physics (particle accelerators), and transportation (magnetic levitation trains). In summary, the historical context of electromagnetism is marked by a series of groundbreaking discoveries that have progressively deepened our understanding of how conductors interact with magnetic fields. From Ørsted's initial observation to the comprehensive theories of Maxwell and the quantum insights of modern physics, each milestone has contributed to our current knowledge and technological capabilities in this field. This rich historical tapestry underscores the importance of continued scientific inquiry and innovation in electromagnetism.
Conditions for a Conductor to Produce a Magnetic Field
The production of a magnetic field by a conductor is a fundamental concept in electromagnetism, underpinning many modern technologies from electrical motors to medical imaging devices. For a conductor to generate a magnetic field, several key conditions must be met. First, the presence of electric current is essential; without it, no magnetic field can be produced. Second, the direction and flow of this current play a crucial role in determining the orientation and strength of the magnetic field. Lastly, the material and shape of the conductor also significantly influence the characteristics of the magnetic field generated. Understanding these conditions is vital for harnessing the power of electromagnetism effectively. In this article, we will delve into each of these critical factors—presence of electric current, direction and flow of current, and influence of conductor material and shape—to provide a comprehensive overview of how conductors produce magnetic fields. By grasping these principles, readers will gain a deeper understanding of the basics of electromagnetism.
Presence of Electric Current
The presence of electric current is a fundamental condition for a conductor to produce a magnetic field. When an electric current flows through a conductor, it generates a magnetic field around the conductor due to the movement of charged particles, typically electrons. This phenomenon is described by Ampère's law, which states that the magnetic field produced by an electric current is directly proportional to the magnitude of the current and inversely proportional to the distance from the conductor. The direction of the magnetic field can be determined using the right-hand rule, where if you point your thumb in the direction of the current flow, your fingers will curl in the direction of the magnetic field. Electric current itself is the flow of electrons through a material, often driven by an external voltage source such as a battery or generator. In conductors like copper wires, electrons are relatively free to move when an electric potential difference is applied across the ends of the wire. This movement of electrons constitutes an electric current, and it is this flow that induces a magnetic field. The strength and orientation of the magnetic field depend on several factors, including the amount of current flowing through the conductor, the cross-sectional area of the conductor, and whether the conductor is straight or coiled. In practical applications, understanding the relationship between electric current and magnetic fields is crucial. For instance, in electrical motors and generators, the interaction between electric currents and magnetic fields enables the conversion of electrical energy into mechanical energy or vice versa. Similarly, in transformers, the magnetic fields produced by alternating currents allow for efficient transfer and transformation of electrical power over long distances. Moreover, the presence of electric current in conductors has significant implications in various technological and scientific contexts. In medical imaging techniques like MRI (Magnetic Resonance Imaging), strong magnetic fields generated by high currents are used to create detailed images of the body's internal structures. In telecommunications, the principles underlying electromagnetic induction are essential for the operation of devices such as antennas and inductors. In summary, the presence of electric current in a conductor is essential for producing a magnetic field. This relationship forms the basis of numerous technological innovations and scientific principles that underpin many modern applications. By understanding how electric currents generate magnetic fields, we can harness these phenomena to develop more efficient and sophisticated technologies that shape our daily lives.
Direction and Flow of Current
When discussing the conditions under which a conductor produces a magnetic field, it is crucial to understand the direction and flow of current. The flow of electric current through a conductor is fundamental in generating a magnetic field. According to Ampère's law, the direction of the magnetic field around a conductor is determined by the direction of the current flow. This relationship is often visualized using the right-hand rule: if you point your thumb in the direction of the current, your fingers will curl in the direction of the magnetic field lines. The flow of current itself is a result of electrons moving within the conductor. In metals, which are common conductors, free electrons are able to move freely under the influence of an electric field. When these electrons flow through a wire, they create an electric current. The magnitude and direction of this current are critical because they directly influence the strength and orientation of the resulting magnetic field. For instance, if current flows through a straight wire, it generates a magnetic field that encircles the wire in concentric circles. The direction of these circles can be determined by observing whether the current is flowing towards or away from an observer. This principle extends to more complex geometries such as coils and solenoids, where the cumulative effect of multiple turns of wire enhances the magnetic field significantly. Moreover, the direction of current flow can be either direct (DC) or alternating (AC). In DC circuits, electrons move in one direction only, resulting in a static magnetic field around the conductor. In contrast, AC circuits involve electrons that oscillate back and forth, producing a magnetic field that also oscillates. This dynamic nature of AC currents is particularly relevant in applications such as transformers and generators where changing magnetic fields are essential for operation. Understanding the direction and flow of current is not only essential for predicting the behavior of magnetic fields but also for designing and optimizing electrical systems. For example, in electrical motors and generators, precise control over current direction ensures efficient conversion between electrical and mechanical energy. Similarly, in telecommunications, knowing how currents flow through conductors helps engineers design cables and transmission lines that minimize signal loss and interference. In summary, the direction and flow of current are pivotal factors in determining when and how a conductor produces a magnetic field. By grasping these principles, engineers can harness the power of electromagnetism to create innovative technologies that underpin modern society. Whether it's powering homes with efficient electrical grids or enabling high-speed data transmission over long distances, understanding current flow is at the heart of it all.
Influence of Conductor Material and Shape
The influence of conductor material and shape on the production of a magnetic field is a critical aspect to consider when examining the conditions under which a conductor generates such a field. The material composition of the conductor significantly affects its ability to produce a magnetic field. Conductors made from materials with high electrical conductivity, such as copper or silver, are more efficient at generating magnetic fields due to their lower resistance to current flow. This is because the magnetic field strength is directly proportional to the current flowing through the conductor. Materials with higher resistivity, like carbon or certain alloys, will produce weaker magnetic fields for the same current due to the increased resistance and subsequent heat dissipation. In addition to material properties, the shape of the conductor also plays a crucial role in determining the magnetic field's characteristics. For instance, a straight wire conductor will produce a magnetic field that encircles the wire in concentric circles, as described by Ampère's law. However, when the conductor is shaped into a coil, the magnetic field becomes more complex and concentrated. In a solenoid (a long, tightly wound coil), the magnetic field inside the coil is nearly uniform and can be significantly stronger than that produced by a straight wire carrying the same current. This is because the fields generated by each turn of the coil add up, creating a cumulative effect that enhances the overall magnetic field strength. Furthermore, the geometry of the conductor can influence the direction and distribution of the magnetic field. For example, a toroidal coil (a doughnut-shaped coil) produces a magnetic field that is confined within the toroid, making it useful in applications where a localized and controlled magnetic environment is required. In contrast, a helical coil can produce a magnetic field that extends beyond its physical boundaries, making it suitable for applications requiring a broader field coverage. Understanding these influences is essential for designing and optimizing electrical devices that rely on magnetic fields, such as motors, generators, transformers, and magnetic resonance imaging (MRI) machines. By carefully selecting the material and shaping the conductor appropriately, engineers can tailor the magnetic field to meet specific requirements, ensuring efficient and effective operation of these devices. Thus, the interplay between conductor material and shape is a fundamental consideration in the study of electromagnetic phenomena and the practical applications thereof.
Practical Applications and Observations
In the realm of physics, the practical applications and observations of magnetic fields are as diverse as they are fascinating. This article delves into the multifaceted world of magnetism, exploring its real-world manifestations, measurement techniques, and industrial uses. We begin by examining real-world examples of magnetic field generation, highlighting how these fields are naturally produced and artificially created in various contexts. Next, we discuss the sophisticated measurement and detection techniques that scientists and engineers employ to quantify and analyze magnetic fields, enabling precise understanding and control. Finally, we explore the industrial and technological uses of magnetism, from medical imaging to high-speed transportation systems, illustrating its critical role in modern technology. By understanding these practical applications and observations, we can better appreciate the underlying principles of electromagnetism, transitioning seamlessly into a deeper exploration of its fundamental concepts. This journey through the practical side of magnetism sets the stage for a comprehensive understanding of the basics of electromagnetism.
Real-World Examples of Magnetic Field Generation
Magnetic fields are ubiquitous in our daily lives, and their generation can be observed in various real-world scenarios. One of the most common examples is the electric motor, which relies on the principle that a current-carrying conductor generates a magnetic field. In an electric motor, when an electric current flows through the coils of the motor, it creates a magnetic field that interacts with the permanent magnets or other magnetic fields within the motor. This interaction causes the motor to rotate, converting electrical energy into mechanical energy. Similarly, generators work on the reverse principle; they convert mechanical energy into electrical energy by rotating a conductor within a magnetic field, inducing an electromotive force (EMF). Another significant example is in medical imaging technology, particularly in Magnetic Resonance Imaging (MRI). In MRI machines, powerful magnetic fields are generated by superconducting magnets to align the hydrogen nuclei in the body. Radiofrequency pulses are then applied to disturb this alignment, and as the nuclei return to their aligned state, they emit signals that are used to create detailed images of internal structures. This process relies on the precise control and generation of strong magnetic fields. In transportation, magnetic fields play a crucial role in high-speed rail systems such as maglev trains. These trains use electromagnets to create magnetic fields that repel and attract each other, allowing the train to float above the track and move at high speeds without physical contact. This technology eliminates friction and significantly reduces wear and tear on the train and track, making it a highly efficient mode of transportation. Additionally, magnetic fields are essential in many consumer electronics. For instance, speakers and headphones use magnetic fields to convert electrical signals into sound waves. When an electric current flows through the coil of a speaker, it generates a magnetic field that interacts with a permanent magnet, causing the diaphragm to vibrate and produce sound. In scientific research, particle accelerators like those at CERN rely heavily on magnetic fields to steer and focus charged particles at incredibly high energies. These magnetic fields are generated by powerful electromagnets that guide the particles along precise trajectories, enabling scientists to study subatomic particles and their interactions in detail. Lastly, in everyday household items like transformers and inductors, magnetic fields are crucial for voltage regulation and energy storage. Transformers use magnetic fields to transfer electrical energy between circuits through electromagnetic induction, while inductors store energy in a magnetic field when current flows through them. These examples illustrate how the generation of magnetic fields by conductors underlies a wide range of practical applications and observations, from industrial machinery and medical imaging to consumer electronics and scientific research. Understanding how conductors produce magnetic fields is fundamental to harnessing their potential in various technological advancements.
Measurement and Detection Techniques
**Measurement and Detection Techniques** Understanding when a conductor produces a magnetic field is crucial for various practical applications, and this knowledge hinges on advanced measurement and detection techniques. These methods are designed to accurately quantify the magnetic fields generated by conductors under different conditions. One of the primary techniques is the use of **Hall Effect sensors**, which measure the voltage generated across a conductor when it is placed in a magnetic field. This voltage, known as the Hall voltage, is directly proportional to the strength of the magnetic field, allowing precise quantification. Another significant technique involves **magnetometers**, highly sensitive devices that detect and measure magnetic fields with great accuracy. These can range from simple analog magnetometers to sophisticated digital ones, such as fluxgate magnetometers or SQUID (Superconducting Quantum Interference Device) magnetometers, which offer unparalleled sensitivity and resolution. For instance, in electrical engineering, magnetometers are used to monitor the magnetic fields around power lines and transformers, ensuring safe and efficient operation. **Current transformers** are also pivotal in measuring the magnetic fields produced by conductors. These devices measure the current flowing through a conductor by detecting the magnetic field it generates, allowing for non-invasive monitoring of electrical currents. This is particularly useful in power distribution systems where real-time current measurement is essential for load management and fault detection. In addition to these tools, **Biot-Savart law** provides a theoretical framework for calculating the magnetic field generated by a conductor. This law relates the magnetic field at any point to the current flowing through the conductor and its geometry, enabling engineers to predict and analyze magnetic field behavior in various scenarios. Furthermore, **magnetic field mapping** techniques involve using arrays of sensors to create detailed maps of magnetic field distributions around conductors. This is particularly useful in research and development, where understanding the spatial distribution of magnetic fields can help optimize device performance and safety. In practical applications, these measurement and detection techniques are integrated into various systems to ensure reliable operation. For example, in medical imaging technologies like MRI (Magnetic Resonance Imaging), precise control over magnetic fields is critical for producing high-resolution images. Similarly, in automotive systems, magnetic field sensors are used in anti-lock braking systems (ABS) to detect wheel speed and prevent skidding. By leveraging these advanced measurement and detection techniques, engineers and scientists can gain deep insights into the behavior of conductors under different conditions, enabling the development of more efficient, safe, and reliable technologies across a wide range of industries. These methods not only enhance our understanding of fundamental physics but also drive innovation in practical applications, making them indispensable tools in modern engineering and research.
Industrial and Technological Uses
Industrial and technological uses of conductors producing magnetic fields are diverse and pivotal, underpinning many modern technologies. In the realm of electrical engineering, conductors are essential for generating magnetic fields in motors, generators, and transformers. Motors, for instance, rely on the interaction between a conductor carrying an electric current and a magnetic field to produce rotational motion, which is crucial in machinery and automotive systems. Generators, on the other hand, convert mechanical energy into electrical energy by exploiting the principle of electromagnetic induction, where a conductor moving within a magnetic field induces an electric current. Transformers utilize conductors to transfer electrical energy efficiently between different voltage levels, enabling the widespread distribution of power across long distances without significant loss. In medical technology, magnetic resonance imaging (MRI) machines depend on powerful magnetic fields generated by conductors to create detailed images of the body's internal structures. These fields are produced by superconducting coils that carry high currents, allowing for precise imaging that aids in diagnosis and treatment planning. Additionally, in materials science and manufacturing, electromagnetic induction heating is used to heat conductive materials rapidly and efficiently, which is particularly useful in processes such as welding and heat treatment. The aerospace industry also leverages conductors producing magnetic fields for navigation and communication systems. Magnetometers, which measure magnetic fields, are used in aircraft and spacecraft to determine orientation and position. Furthermore, radio frequency (RF) coils in communication systems generate magnetic fields to transmit and receive signals over long distances. In consumer electronics, conductors producing magnetic fields are integral to devices like speakers and headphones. The movement of a conductor within a magnetic field converts electrical signals into sound waves, enabling audio reproduction. Similarly, in data storage technology, hard disk drives use conductors to read and write data on magnetic disks, facilitating high-capacity data storage. Moreover, in renewable energy systems, conductors are used in wind turbines and hydroelectric generators to convert mechanical energy into electrical energy through electromagnetic induction. This technology is vital for harnessing sustainable energy sources efficiently. In summary, the practical applications of conductors producing magnetic fields span a wide range of industries and technologies, from power generation and distribution to medical imaging, materials processing, aerospace navigation, consumer electronics, and renewable energy systems. These applications underscore the fundamental importance of understanding when and how conductors produce magnetic fields in various contexts.