Where Does Pyruvate Oxidation Occur
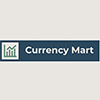
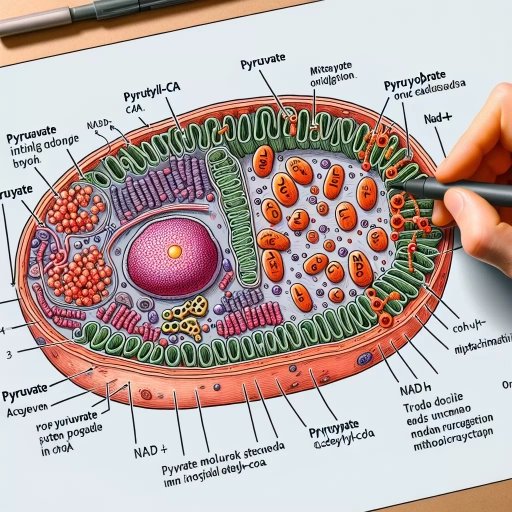
Pyruvate oxidation is a crucial metabolic process that bridges glycolysis and the citric acid cycle, playing a pivotal role in cellular energy production. This intricate biochemical pathway not only converts pyruvate into acetyl-CoA but also sets the stage for further oxidative phosphorylation. Understanding where pyruvate oxidation occurs within the cell is essential for grasping its significance in cellular metabolism. The location of pyruvate oxidation, specifically within the mitochondria, highlights its strategic position in energy production. Additionally, delving into the molecular mechanisms involved reveals a complex interplay of enzymes and coenzymes that facilitate this conversion. This article will explore these aspects comprehensively, starting with an introduction to pyruvate oxidation.
Introduction to Pyruvate Oxidation
Pyruvate oxidation is a critical biochemical process that plays a pivotal role in cellular respiration, the primary mechanism by which cells generate energy. This intricate process involves the conversion of pyruvate, a product of glycolysis, into acetyl-CoA, which then enters the citric acid cycle to produce ATP. Understanding pyruvate oxidation is essential for comprehending how cells efficiently utilize glucose to produce energy. In this article, we will delve into the definition and importance of pyruvate oxidation, exploring its fundamental role in cellular metabolism and why it is crucial for maintaining cellular homeostasis. We will also examine the historical context and discovery of this process, highlighting key milestones and scientists who contributed to our current understanding. Finally, we will discuss the role of pyruvate oxidation in cellular respiration, detailing how it integrates with other metabolic pathways to ensure optimal energy production. By exploring these aspects, we aim to provide a comprehensive introduction to pyruvate oxidation.
Definition and Importance of Pyruvate Oxidation
Pyruvate oxidation is a critical biochemical process that occurs within the mitochondria of cells, marking the transition from glycolysis to the citric acid cycle. This process involves the conversion of pyruvate, a three-carbon molecule produced during glycolysis, into acetyl-CoA. This conversion is catalyzed by the pyruvate dehydrogenase complex (PDC), a multi-enzyme complex located in the mitochondrial matrix. The importance of pyruvate oxidation cannot be overstated; it serves as a pivotal step in cellular respiration, allowing cells to efficiently generate energy from glucose. The significance of pyruvate oxidation lies in its role as a gateway to the citric acid cycle and subsequent oxidative phosphorylation. By converting pyruvate into acetyl-CoA, cells can feed this molecule into the citric acid cycle, where it undergoes further oxidation and produces NADH and FADH2. These electron carriers then donate their electrons to the electron transport chain, driving ATP synthesis through oxidative phosphorylation. This process is essential for aerobic organisms because it maximizes energy yield from glucose compared to anaerobic processes like lactic acid fermentation or ethanol fermentation. Moreover, pyruvate oxidation plays a crucial role in regulating cellular metabolism. It acts as a key regulatory point that integrates signals from various metabolic pathways to ensure balanced energy production and consumption. For instance, high levels of ATP inhibit PDC activity, thereby reducing pyruvate oxidation when energy demands are low. Conversely, low ATP levels activate PDC to increase pyruvate oxidation and boost energy production. In addition to its central role in energy metabolism, pyruvate oxidation has implications for cellular health and disease. Dysregulation or defects in this pathway have been linked to various pathologies such as neurodegenerative diseases like Alzheimer's and Parkinson's disease, where impaired mitochondrial function leads to reduced energy availability for neurons. Similarly, cancer cells often exhibit altered pyruvate metabolism due to mutations or changes in gene expression that favor anaerobic glycolysis over oxidative phosphorylation—a phenomenon known as the Warburg effect. In conclusion, pyruvate oxidation is an indispensable process that bridges glycolysis with the citric acid cycle and oxidative phosphorylation. Its importance extends beyond mere energy production; it also plays a critical role in regulating cellular metabolism and maintaining cellular health. Understanding this process is vital for appreciating how cells manage their energy resources efficiently and how disruptions can lead to disease states.
Historical Context and Discovery
The discovery of pyruvate oxidation is deeply rooted in the historical context of cellular respiration research. In the early 20th century, scientists began to unravel the mysteries of how cells generate energy. The pioneering work by Otto Meyerhof and Archibald Hill in the 1920s laid foundational insights into glycolysis and oxidative phosphorylation. Meyerhof's experiments with muscle tissue revealed that glycolysis produces pyruvate, which then undergoes further oxidation to produce ATP. This breakthrough was pivotal as it connected glycolysis with the subsequent steps of cellular respiration. In the following decades, researchers like Hans Krebs and Fritz Lipmann further elucidated these processes. Krebs' discovery of the citric acid cycle (also known as the Krebs cycle or tricarboxylic acid cycle) in 1937 provided a crucial link between pyruvate oxidation and ATP production. Lipmann's work on coenzyme A (CoA) in the 1940s explained how pyruvate is converted into acetyl-CoA, a key intermediate that feeds into the citric acid cycle. The 1950s saw significant advancements with the identification of mitochondria as the primary site for pyruvate oxidation. Electron microscopy revealed mitochondria's unique structure, including cristae that increase surface area for oxidative reactions. This structural insight supported biochemical findings that mitochondria house both the citric acid cycle and electron transport chain—essential components for converting pyruvate into ATP. Modern research has continued to refine our understanding through advanced techniques such as nuclear magnetic resonance spectroscopy (NMR) and mass spectrometry. These tools allow scientists to trace metabolic pathways in real-time, providing detailed insights into how pyruvate is metabolized under various physiological conditions. Understanding where pyruvate oxidation occurs is essential for appreciating its role in cellular metabolism. Pyruvate oxidation takes place within mitochondria, specifically within their matrix where enzymes catalyze its conversion to acetyl-CoA. This process is tightly regulated by feedback mechanisms involving ATP levels and other metabolic intermediates. In summary, historical discoveries have progressively illuminated our knowledge about pyruvate oxidation from initial biochemical observations to detailed structural insights into mitochondrial function. Today’s sophisticated analytical techniques continue to enhance our comprehension of this critical metabolic pathway.
Role in Cellular Respiration
Cellular respiration is a critical metabolic process that converts glucose into energy in the form of ATP. This intricate process involves several stages, including glycolysis, pyruvate oxidation, the Krebs cycle, and oxidative phosphorylation. Pyruvate oxidation plays a pivotal role in bridging glycolysis and the Krebs cycle by converting pyruvate into acetyl-CoA. This conversion occurs within the mitochondria, specifically in the mitochondrial matrix. The enzyme pyruvate dehydrogenase complex catalyzes this reaction, which involves the decarboxylation of pyruvate and its subsequent conversion into acetyl-CoA. This step is crucial because acetyl-CoA then enters the Krebs cycle (also known as the citric acid cycle), where it undergoes further oxidation to produce NADH and FADH2 as byproducts. These electron carriers are essential for generating ATP during oxidative phosphorylation in the electron transport chain. Pyruvate oxidation is tightly regulated by various mechanisms to ensure that energy production is balanced with cellular needs. For instance, high levels of ATP inhibit pyruvate dehydrogenase activity to prevent unnecessary energy expenditure when cellular ATP levels are sufficient. Conversely, low ATP levels activate this enzyme to increase acetyl-CoA production and subsequently boost energy production through the Krebs cycle and oxidative phosphorylation. In summary, pyruvate oxidation is a vital link between glycolysis and subsequent stages of cellular respiration. Its occurrence within mitochondria highlights its central role in energy metabolism, making it an indispensable component for maintaining cellular homeostasis and supporting life processes.
Location of Pyruvate Oxidation in the Cell
Pyruvate oxidation is a critical metabolic process that converts pyruvate, the end product of glycolysis, into acetyl-CoA, which then enters the citric acid cycle to produce ATP. This process is essential for cellular energy production and occurs primarily in the mitochondrial matrix. The mitochondrial matrix serves as the primary site for pyruvate oxidation due to its high concentration of necessary enzymes and cofactors. Additionally, mitochondrial transport mechanisms play a crucial role in facilitating the movement of pyruvate and other substrates across the mitochondrial membrane, ensuring efficient oxidation. Comparatively, other cellular compartments lack the specialized environment and machinery required for efficient pyruvate oxidation. Understanding these aspects provides comprehensive insights into how cells optimize energy production through pyruvate oxidation. This article will delve into these key concepts to offer a detailed exploration of where and how pyruvate oxidation occurs within cells. Introduction to Pyruvate Oxidation
Mitochondrial Matrix as the Primary Site
The mitochondrial matrix is the primary site where pyruvate oxidation occurs within the cell. This process is crucial for cellular respiration, as it converts pyruvate, a product of glycolysis, into acetyl-CoA, which then enters the citric acid cycle (also known as the Krebs cycle or tricarboxylic acid cycle). The mitochondrial matrix provides an optimal environment for this conversion due to its high concentration of enzymes and coenzymes necessary for these reactions. Specifically, pyruvate dehydrogenase complex (PDC), a multi-enzyme complex located in the mitochondrial matrix, catalyzes the conversion of pyruvate to acetyl-CoA. This complex requires coenzymes such as thiamine pyrophosphate (TPP), lipoic acid, CoA-SH, FAD, and NAD+, which are abundantly available in this region. The close proximity of these components ensures efficient transfer of intermediates and electrons during oxidative decarboxylation. Moreover, the mitochondrial matrix is rich in ATP synthase and other electron transport chain components that utilize electrons from NADH and FADH2 produced during pyruvate oxidation to generate ATP through oxidative phosphorylation. This localized arrangement maximizes energy yield by minimizing diffusion distances and ensuring rapid utilization of reducing equivalents. Additionally, regulatory mechanisms such as feedback inhibition by ATP/ADP ratios and NAD+/NADH ratios are finely tuned within this compartment to control metabolic flux according to cellular energy demands. In summary, the mitochondrial matrix serves as an ideal locale for pyruvate oxidation due to its unique biochemical environment that facilitates efficient enzymatic activity and energy production. Its role underscores why mitochondria are often referred to as "the powerhouses" of cells—highlighting their critical function in converting metabolic substrates into usable energy through oxidative processes.
Role of Mitochondrial Transport Mechanisms
Mitochondrial transport mechanisms play a crucial role in the process of pyruvate oxidation, ensuring that this essential metabolic pathway functions efficiently. Pyruvate, the end product of glycolysis, must be transported into the mitochondria to undergo oxidative phosphorylation. This transport is facilitated by specific transporters embedded in the mitochondrial membranes. The monocarboxylate transporters (MCTs), particularly MCT1 and MCT4, are key players in this process. They shuttle pyruvate across the plasma membrane and into the cytoplasm, where it can then be taken up by mitochondria through another set of transporters. Once inside the mitochondria, pyruvate is converted into acetyl-CoA by pyruvate dehydrogenase (PDH), a multi-enzyme complex located within the mitochondrial matrix. This conversion is critical as it links glycolysis to the citric acid cycle (Krebs cycle), allowing for further energy production through oxidative phosphorylation. The efficiency of this conversion process depends on various factors including coenzyme availability and redox state within the mitochondria. Mitochondrial transport mechanisms also regulate other essential metabolites involved in pyruvate oxidation. For instance, carnitine palmitoyltransferase I (CPT-I) facilitates fatty acid transport into mitochondria for beta-oxidation, which can influence pyruvate metabolism indirectly by affecting energy demand and redox balance within cells. Moreover, mitochondrial dynamics—such as fission and fusion—play a significant role in maintaining optimal mitochondrial function during periods of high energy demand or stress conditions where efficient pyruvate oxidation is crucial for cellular survival. In summary, mitochondrial transport mechanisms are indispensable for ensuring that pyruvate reaches its site of oxidation within mitochondria efficiently. These mechanisms not only facilitate direct transport but also maintain cellular homeostasis necessary for optimal metabolic function during various physiological states or stress conditions.
Comparison with Other Cellular Compartments
When comparing the location of pyruvate oxidation to other cellular compartments, it becomes evident that this process is uniquely situated within the mitochondria. Unlike glycolysis, which occurs in the cytoplasm and can function anaerobically, pyruvate oxidation is an aerobic process that requires oxygen and takes place in the mitochondrial matrix. This compartmentalization is crucial because it allows for efficient energy production through oxidative phosphorylation. The mitochondria's double membrane structure and cristae provide a large surface area for electron transport chains to operate, maximizing ATP yield from each glucose molecule. In contrast, the endoplasmic reticulum (ER) is primarily involved in protein synthesis and lipid metabolism. While it does have some enzymatic functions, it does not play a role in pyruvate oxidation. Similarly, lysosomes are specialized for degradation and recycling of cellular components but are not involved in energy production pathways like pyruvate oxidation. The Golgi apparatus modifies proteins and lipids synthesized by the ER but does not participate in metabolic processes such as pyruvate oxidation. The peroxisomes are another type of organelle that deal with oxidative reactions; however, their primary function is to break down fatty acids and amino acids through beta-oxidation rather than handling pyruvate directly. Chloroplasts in plant cells are responsible for photosynthesis but do not engage in pyruvate oxidation as part of their metabolic processes. The nucleus houses genetic material and regulates gene expression but does not directly participate in metabolic pathways like pyruvate oxidation. Cytosol serves as a medium for various metabolic reactions including glycolysis; however, once glycolysis converts glucose into pyruvate, this molecule must be transported into mitochondria for further oxidation. In summary, while different cellular compartments have distinct roles essential for overall cellular function—such as protein synthesis (ER), degradation (lysosomes), modification (Golgi apparatus), fatty acid breakdown (peroxisomes), photosynthesis (chloroplasts), genetic regulation (nucleus), and initial glucose metabolism (cytosol)—the mitochondria stand out as the exclusive site where pyruvate is oxidized to produce ATP efficiently through oxidative phosphorylation. This specialized localization underscores the critical importance of mitochondrial function in cellular energy metabolism.
Molecular Mechanisms Involved in Pyruvate Oxidation
Pyruvate oxidation is a critical metabolic process that bridges glycolysis and the citric acid cycle, ensuring efficient energy production in cells. This intricate pathway involves several key molecular mechanisms that orchestrate the conversion of pyruvate into acetyl-CoA, a pivotal step for cellular respiration. Central to this process is the Pyruvate Dehydrogenase Complex (PDC), a multi-enzyme complex that catalyzes the decarboxylation of pyruvate. Coenzyme A (CoA) and NAD+ play essential roles as cofactors, facilitating the transfer of acetyl groups and electrons, respectively. Additionally, pyruvate oxidation is tightly regulated by feedback inhibition and allosteric control mechanisms to ensure metabolic homeostasis. Understanding these molecular mechanisms provides valuable insights into cellular metabolism and has significant implications for various physiological and pathological processes. This article delves into these critical aspects, offering a comprehensive overview of the molecular intricacies involved in pyruvate oxidation. Introduction to Pyruvate Oxidation
Pyruvate Dehydrogenase Complex (PDC)
Pyruvate Dehydrogenase Complex (PDC) is a pivotal enzyme complex in the molecular mechanisms involved in pyruvate oxidation. Located in the mitochondrial matrix, PDC catalyzes the conversion of pyruvate, a product of glycolysis, into acetyl-CoA, which then enters the citric acid cycle (Krebs cycle) for further oxidation. This process is crucial for cellular respiration and energy production. The PDC complex consists of three main components: pyruvate dehydrogenase (E1), dihydrolipoyl transacetylase (E2), and dihydrolipoyl dehydrogenase (E3). Each component plays a specific role in the multi-step reaction that ultimately leads to the formation of acetyl-CoA. The first step involves E1, which decarboxylates pyruvate to form a hydroxyethyl group attached to thiamine pyrophosphate (TPP). This intermediate is then transferred to lipoic acid on E2, where it undergoes an oxidation reaction that results in the formation of acetyl-CoA. The reduced lipoic acid is subsequently reoxidized by E3, which uses NAD+ as an electron acceptor, producing NADH as a byproduct. This NADH can contribute to ATP synthesis through oxidative phosphorylation. Regulation of PDC activity is tightly controlled by various mechanisms to ensure efficient energy metabolism. Phosphorylation-dephosphorylation cycles mediated by pyruvate dehydrogenase kinase (PDK) and pyruvate dehydrogenase phosphatase (PDP) play significant roles. PDK inhibits PDC activity by phosphorylating it, while PDP activates it by dephosphorylating it. Additionally, allosteric regulation by metabolites such as ATP, NADH, and acetyl-CoA provides feedback control based on cellular energy status. Dysregulation or mutations affecting PDC components can lead to metabolic disorders and diseases like pyruvate dehydrogenase deficiency, which often manifest with neurological symptoms due to impaired energy supply in neurons. Understanding these molecular mechanisms not only sheds light on normal cellular function but also provides insights into potential therapeutic targets for treating related pathologies. In summary, Pyruvate Dehydrogenase Complex is central to pyruvate oxidation within mitochondria, facilitating the conversion of pyruvate into acetyl-CoA through a series of enzyme-catalyzed reactions involving E1, E2, and E3 components. Its regulation ensures optimal energy production according to cellular needs and highlights its importance in maintaining metabolic homeostasis.
Coenzyme A and NAD+ Involvement
Pyruvate oxidation is a critical metabolic process that occurs in the mitochondria, where pyruvate, the end product of glycolysis, is converted into acetyl-CoA. This conversion is facilitated by the pyruvate dehydrogenase complex (PDC), a multi-enzyme complex located in the mitochondrial matrix. Coenzyme A (CoA) and NAD+ play pivotal roles in this process. CoA, a thiol-containing coenzyme, acts as a carrier molecule for acetyl groups. It binds to pyruvate, forming acetyl-CoA, which then enters the citric acid cycle (Krebs cycle). This step is essential for generating energy through cellular respiration. NAD+, or nicotinamide adenine dinucleotide, is another crucial coenzyme involved in pyruvate oxidation. During the conversion of pyruvate to acetyl-CoA by PDC, NAD+ serves as an electron acceptor. It gets reduced to NADH while donating electrons that are subsequently passed through the electron transport chain in the mitochondrial inner membrane. This electron transport generates ATP via oxidative phosphorylation, contributing significantly to cellular energy production. The involvement of CoA and NAD+ ensures efficient energy metabolism by linking glycolysis with oxidative phosphorylation. Their roles highlight how tightly regulated and interconnected metabolic pathways are essential for maintaining cellular homeostasis and supporting various physiological functions. Understanding these molecular mechanisms provides insights into how cells manage their energy needs and how dysregulation can lead to metabolic disorders or diseases such as diabetes and neurodegenerative conditions. In summary, CoA and NAD+ are indispensable components of pyruvate oxidation within mitochondria. Their precise interactions facilitate not only the conversion of pyruvate into acetyl-CoA but also drive forward cellular respiration's energy-producing processes. This intricate biochemical interplay underscores why these coenzymes are central to understanding both normal metabolic function and potential therapeutic targets for treating related pathologies.
Regulation by Feedback Inhibition and Allosteric Control
Regulation by feedback inhibition and allosteric control plays a crucial role in the molecular mechanisms involved in pyruvate oxidation. This regulatory process ensures that the metabolic pathway is finely tuned to meet the cell's energy demands while preventing unnecessary or excessive activity. Feedback inhibition involves the end product of a metabolic pathway inhibiting an earlier step, thereby preventing overproduction. In pyruvate oxidation, ATP and NADH serve as key regulators; when their levels are high, they signal that energy is abundant, leading to reduced activity in the pathway. Conversely, when ATP and NADH levels are low, indicating a need for more energy production, the pathway is activated. Allosteric control involves the binding of regulatory molecules to specific sites on enzymes, altering their activity without affecting their active sites directly. This mechanism allows for rapid adjustments in response to changing cellular conditions. For example, pyruvate dehydrogenase (PDH), the enzyme responsible for converting pyruvate into acetyl-CoA, is allosterically inhibited by ATP and NADH but activated by ADP and NAD+. This dual regulation ensures that PDH activity is precisely controlled based on cellular energy status. Additionally, covalent modifications such as phosphorylation/dephosphorylation cycles further modulate PDH activity. The pyruvate dehydrogenase kinase (PDK) phosphorylates PDH subunits, rendering it inactive when ATP levels are high. Conversely, pyruvate dehydrogenase phosphatase (PDP) dephosphorylates PDH subunits when ATP levels drop, reactivating it. This dynamic interplay between allosteric regulation and covalent modification provides a robust mechanism for maintaining homeostasis during pyruvate oxidation. Moreover, other factors such as Ca2+ ions also play a role in regulating PDH activity through allosteric mechanisms. Increased intracellular Ca2+ levels signal increased energy demand during muscle contraction or neuronal activity; this leads to activation of PDH via direct binding or through activation of PDP. In summary, regulation by feedback inhibition and allosteric control is essential for maintaining efficient and balanced pyruvate oxidation within cells. These mechanisms ensure that energy production is tightly regulated according to cellular needs while preventing wasteful overproduction or underproduction of metabolic intermediates.